the Creative Commons Attribution 4.0 License.
the Creative Commons Attribution 4.0 License.
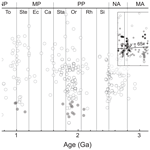
Some thoughts about eclogites and related rocks
The past 40 years have been a golden age for eclogite studies, supported by an ever wider range of instrumentation and enhanced computational capabilities, linked with ongoing developments in thermobarometry and geochronology. During this time, we have made robust estimates of pressure–temperature (P–T) conditions; determined ages related to the prograde, metamorphic peak and retrograde stages; and calculated time-integrated rates of cooling and exhumation for eclogites and related rocks, including blueschists, from orogenic belts worldwide. Improvements to single mineral thermometers and new developments in elastic barometry using inclusions of one mineral in another (e.g. quartz and/or zircon in garnet), coupled with ongoing innovations in petrochronology and diffusion modelling, presage a new age for eclogite studies in which detailed quantification of metamorphic conditions and timescales will be linked to an improved understanding of processes at all scales. Since the turn of the century, numerical modelling of subduction zone and rock exhumation processes has become increasingly important. As a result, subduction and exhumation are quite well understood, but the volume of continental crust subducted to and returned from mantle conditions and the amount lost to the mantle are largely unknown. We have generated sufficient data to investigate the spatiotemporal distribution of metamorphism and secular change but not without controversy in relation to the rare occurrence of orogenic eclogites and the absence of blueschists prior to the late Neoproterozoic and the emergence of plate tectonics on Earth. Since the turn of the century, the assumption that metamorphic pressure is lithostatic has come under increasing scrutiny. Whether local variations in stress extrapolate to the crustal scale and, if so, whether the magnitude of the calculated deviations from lithostatic pressure can be generated and sustained in mechanically heterogeneous rock units remains contentious. Could the paradigm of subduction of continental lithosphere to mantle depths be simply an artefact of the lithostatic assumption? Fluid cycling in subduction zones and understanding the role of fluids in the generation of intermediate-depth earthquakes remain important topics of current research. Dry (H2O-absent) conditions are unlikely around the peak of ultrahigh-pressure (UHP) metamorphism or during exhumation, due to dehydroxylation of nominally anhydrous minerals and breakdown of hydrous minerals at P–T conditions in the realm of supercritical fluid and hydrous melt. Indeed, the presence of melt may be necessary to facilitate the exhumation of HP and UHP tectonometamorphic rock units. Finally, our ability to interrogate inclusions in superdeep diamonds should lead to a better understanding of how the deep interior and surface are linked in the context of Earth as a fully coupled system.
- Article
(775 KB) - Full-text XML
- BibTeX
- EndNote
Eclogites generally represent metamorphosed basic rocks – basalts and gabbros. Their simple mineralogy – dominantly garnet and omphacite, with one or more of kyanite, rutile, quartz/coesite, orthopyroxene, lawsonite, amphibole, phengite/paragonite or epidote/zoisite – belies the many significant advances in our understanding of tectonic processes that have been derived from the study of orogenic eclogites. The history of eclogite during the nineteenth and twentieth centuries, both the advances in our petrological knowledge and its role in geodynamics, has been reviewed by Godard (2001). For each topic discussed below, I provide a brief historical context, review our progress as a community in quantifying the intensive variables and in understanding some of the fundamental processes pertaining to eclogite metamorphism in relation to orogenesis, and offer some suggestions for future research.
Following the paradigm shift from geosynclinal theory to plate tectonics in the late 1960s (Dewey and Bird, 1970) and the demonstration that the jadeite–glaucophane type of regional metamorphism could be related to subduction and plate tectonics in the early 1970s (Ernst, 1971, 1973), evidence began to emerge of unusually high pressures (at the time) associated with subducted rocks. For example, metamorphic conditions of 700–850 ∘C and 3.0–4.5 GPa were retrieved from country rock orthopyroxene eclogite pods in basement gneisses from western Norway, which, in the absence of evidence of similar pressures in the host gneisses, were interpreted as tectonically emplaced exotic inclusions (Lappin and Smith, 1978). In the following year, broadly similar conditions of 800 ∘C and 2.5 GPa were reported for metarodingites from the Swiss Alps (Evans et al., 1979).
The first International Eclogite Conference was held in 1982 and was followed by an age of spectacular discoveries. First, coesite was found in crustal eclogite from the Italian Alps (Chopin, 1984) and the Norwegian Caledonides (Smith, 1984), demonstrating that metamorphic pressures at these localities had exceeded ∼ 2.7 GPa. Then, microdiamond was identified as inclusions in garnet in high-pressure metamorphic garnet–pyroxene and pyroxene–carbonate–garnet rocks, as well as garnet–biotite gneisses and schists from the Kokchetav Massif in northern Kazakhstan (Sobolev and Shatsky, 1990), indicating pressures of crustal metamorphism there were likely > 4.0 GPa. Subsequently, supersilicic (majoritic) garnet was found in orogenic peridotite (van Roermund and Drury, 1998), and supersilicic titanite was discovered in marble from the Kokchetav Massif (Ogasawara et al., 2002), each of which imply pressures > 6 GPa. In theory, these pressures are close to the point of no return for the bulk of the continental crust, and instances of rocks returned from well beyond this limit require exceptional exhumation dynamics or incomplete transformation to an ultrahigh-pressure mineralogy (Chapman et al., 2019). Notwithstanding, quartz paramorphs after stishovite have been identified in an eclogite from the South Altyn Tagh in western China, suggesting exhumation from a minimum pressure of > 8–9 GPa at 800–1000 ∘C (Liu et al., 2018). During the 1980s the term ultrahigh-pressure (UHP) metamorphism was introduced for metamorphic conditions above the coesite–quartz transition. Today the eclogite facies is generally split into the high-pressure (HP) quartz eclogite and the UHP coesite eclogite facies, with the latter sometimes split again to include a diamond eclogite facies at the highest pressures of crustal metamorphism.
The discoveries described above have come from collisional orogens, which were an early focus of research on HP and, particularly, UHP metamorphism. However, it is important to distinguish between episodic attempts to subduct the continental lithosphere during collisional orogenesis and the continuous subduction of the ocean lithosphere as the main driving force of plate tectonics. The latter leads to the disappearance of almost all the ocean lithosphere back into the mantle, although during ocean plate subduction HP metamorphic rocks can be detached and exhumed episodically along the strike of a given trench related to processes such as changes in plate kinematics or transient events such as the subduction of asperities on the downgoing plate (Agard et al., 2009). Some slivers of HP blueschist and eclogite that occur in orogenic sutures represent fragments of the ocean lithosphere trapped during collisional orogenesis. These rocks are distinct from the HP–UHP eclogites intimately associated with felsic gneisses that represent the extended continental lithosphere of a passive margin as it was subducted during terminal collision. By contrast with these continental eclogites, the oceanic eclogites and related rocks appear to have been returned from maximum pressures of less than ∼ 2.8 GPa (Agard et al., 2018). Processes related to the subduction and obduction of ocean lithosphere during the Phanerozoic were reviewed earlier this year by Agard et al. (2023), and the processes by which subduction zones formed during the Cenozoic, from initiation to self-sustained subduction, were reviewed recently by Lallemand and Arcay (2021); consequently, these processes are not considered in detail herein. Lastly, I include only brief comments about xenolithic eclogites, where essential to the discussion, because they also are the subject of a recently published review (Aulbach and Smart, 2023).
I begin with a summary of the methods used to quantify temperature (T), pressure (P) and age (time, t) because these data are fundamental to all research in metamorphic petrology. I briefly review contributions based on numerical modelling of subduction zone processes, an approach that has come to the fore during the past 20 years that will become an important method of testing new ideas in the future as the models become ever more sophisticated. Robust P–T–t data allow us to address fundamental questions, such as how the spatiotemporal distribution of metamorphism relates to convergent plate boundaries and the supercontinent cycle and how the metamorphic record relates to the stabilization of subduction and the emergence and evolution of plate tectonics on Earth. Next, I consider the long-standing controversy over whether metamorphic pressures are equivalent to the lithostatic load (overburden) or whether calculated pressures include a component of tectonic overpressure such that variations in metamorphic pressure from one lithology or tectonic unit to another are unrelated to overburden thickness. Many eclogite protoliths (metabasic rocks) include hydrous minerals during the early stages of their prograde metamorphism, and since eclogites most commonly relate to subduction of oceanic and continental lithosphere, there is an important relationship between subduction and fluid cycling. I briefly address the interplay between mineral reactions, fluid generation and earthquakes. As temperature increases with depth during subduction there is a transition from aqueous fluid to hydrous melt at HP conditions and from hydrous melt to supercritical fluid at UHP conditions so that at maximum P–T conditions and during exhumation supercritical fluid and/or melt is commonly present, although how the fluid/melt was generated remains largely speculative. Lastly, I briefly review information retrieved from inclusion suites in superdeep diamonds, which are derived from below the continental lithosphere and most likely from the transition zone or the top of the lower mantle. These diamonds have provided evidence for transport of surface fluids into the lower mantle, including the possible relationship between fluids and deep earthquakes. For most of these topics, I begin with a brief summary of key advances, continue with a discussion of the main research questions currently being addressed by the community and finish with some comments about the future direction of research.
Currently, the community has catalogued more than 70 localities with reliable ages that record UHP metamorphic conditions (Brown et al., 2022). Many of these localities have been identified based on thermobarometry rather than the presence of coesite or diamond, which is important given the possibility that quartz included in garnet along the prograde P–T path may fail to convert to coesite (Alvaro et al., 2020; Campomenosi et al., 2023b). There are 17 localities where diamond has been recognized, 16 in Phanerozoic collisional orogens and 1 in the Paleoproterozoic Nagssugtoqidian orogen (Dobrzhinetskaya et al., 2022). Much of the recent dating has followed the newly developed methods of petrochronology (Kohn et al., 2017). In an important advance during the last few years, HP and UHP metamorphism has been confirmed at some localities based on detrital garnets and their mineral inclusions (Schönig et al., 2022). As a result, our community has enjoyed a golden age of quantification of P–T, developed better links between P–T and age, and made estimates of time-integrated rates of cooling and exhumation. This section summarizes the state of the art and likely future advances.
2.1 Thermobarometry
Since the 1960s, the range of instrumentation for analysis and characterization of materials and the available computing power have expanded beyond imagination. These advances have enabled us to retrieve information from rocks about their evolution through an orogenic cycle, principally variations in P and T during burial and heating and exhumation and cooling, and the rate of change based on geochronology and diffusion chronometry. These data are commonly summarized as paths annotated with age in P–T space.
Recrystallization of protolith rocks is driven by the need to evolve towards thermodynamic equilibrium as the intensive variables (mostly P, T and chemical potential) change during subduction and orogenesis. Thermobarometry relies on an assumption of equilibrium, whether based on the inversion of mineral compositions to determine P–T values or forward modelling of phase relations for a particular composition to determine the P–T field for an inferred equilibrium phase assemblage. The evolution of computational methods and tools for thermodynamic modelling began with a multiple-reaction approach to thermobarometry in the late 1980s (e.g. de Capitani and Brown, 1987; Berman, 1988, 1991; Powell and Holland, 1988, 1994) before moving on to the calculation of phase diagrams either to show equilibrium assemblages in P–T space for a specific composition or to show equilibrium assemblages in relation to P or T versus a compositional variable (Connolly, 1990, 2005; Powell et al., 1998; Powell and Holland, 2008; de Capitani and Petrakakis, 2010). Calculated phase diagrams include the constraint of composition of the system, generally using either the bulk rock composition or the local reactive bulk composition, where the later may change along the P–T evolution due to compositional fractionation. This constraint sometimes may be useful at higher variances as commonly occurs with eclogite phase assemblages, where the minerals within an equilibrium phase assemblage may show little change in composition over a wide range of P and T but the phase assemblage field may be restricted to a small range of P and T (Powell and Holland, 2008). However, our community must avoid falling into the trap of an uncritical application of calculated phase diagrams based on a belief that phase equilibria can be reliably predicted. Many of the problems inherent in thermodynamic modelling of metamorphic phase assemblages have been discussed by Palin et al. (2016) and Lanari and Engi (2017), to which the interested reader is referred for more detailed information. Importantly, advances in single-element thermometry and elastic thermobarometry (discussed below) allow us to check P–T estimates from phase equilibrium modelling by looking for compatibility with results from these independent methods.
Evaluation of open-system processes, such as the effects of melt loss, have been investigated using a stepwise approach, for example, by reintegrating melt into a residual bulk chemical composition along a schematic P–T path to recover a plausible protolith composition (e.g. Korhonen et al., 2013). However, with the introduction of the free software tool Rcrust, which performs phase stability calculations with path dependence, a reactive system can now be modelled with automated handling of compositional changes as a result of phase addition or removal, including melt loss (Mayne et al., 2016). In addition, the last few years have seen the development of sophisticated software to undertake calculations based on iterative thermodynamic modelling (Duesterhoeft and Lanari, 2020; Xiang and Connolly, 2022) and its application to rocks that preserve microstructural evidence of overprinting to trace sequential partial equilibrium relationships and reconstruct P–T paths (Lanari and Hermann, 2021).
Partly in parallel with advances in thermodynamic modelling, we have seen the development of single-element thermometry and elastic barometry. The main single-element thermobarometers are Ti in zircon and Zr in rutile (Ferry and Watson, 2007), Ti in quartz (Thomas et al., 2010), Ti in phengite (Auzanneau et al., 2010), and Si in phengite (Hermann, 2003). The Zr in rutile and Ti in quartz thermobarometers have been improved over the years (e.g. Tomkins et al., 2007; Kohn, 2020; Osborne et al., 2022). However, whether these thermobarometers record close-to-peak metamorphic temperatures during subduction at blueschist and quartz eclogite facies P–T conditions is an open question that should be evaluated on a study-by-study basis and was demonstrably not the case in at least two case studies to date (Ashley et al., 2013; Spear et al., 2023).
Estimating the P–T conditions of crystallization from the elastic strain developed around inclusions of one mineral in another due to differential volume change during exhumation has a long history extending back to the proposal by Rosenfeld and Chase (1961). Nevertheless, it is only during the past decade that increased access to Raman spectroscopy; advances in mineral physics theory; and new measurements of the elastic properties of minerals, although unfortunately still not at the elevated P–T conditions of the eclogite facies, have led to the rapid development and application of elastic thermobarometry (Angel et al., 2014, 2017, 2019; Bonazzi et al., 2022; Murri et al., 2018; Cisneros and Befus, 2020; Gilio et al., 2022; Kohn et al., 2023). The method was originally based on the assumption of an apparently linear dependency between observed changes in Raman peak positions and the pressure developed in quartz inclusions in garnet during exhumation and cooling due to differences in their compressibilities and thermal expansivities (Enami et al., 2007; Kohn, 2014; Thomas and Spear, 2018). However, in detail this assumption has been shown to be incorrect, with the dependency related to the imposed strain rather than the pressure (Murri et al., 2018; Gilio et al., 2021; Kohn et al., 2023). Thus, the P–T conditions at entrapment should be determined based on measurements of the residual strains that develop in mineral inclusions in a host grain, for example quartz or zircon in garnet or quartz in zircon, due to the differential volume change during exhumation (Gilio et al., 2021; Mazzucchelli et al., 2021; Murri et al., 2022).
The match between the volume of the inclusion and the host is dependent on P–T, generating an isomeke (a line in P–T space along which there is no mismatch in volume between inclusion and host) but varies with mineralogy (Angel et al., 2014). Thus, the use of more than one inclusion type in a common host (e.g. quartz and zircon inclusions in garnet) allows for the determination of both P and T from intersecting isomekes (Spránitz et al., 2023). However, since the assumption of elastic behaviour may be invalidated during close-to-isothermal exhumation, UHP metamorphic rocks in particular may record a pressure related to resetting during exhumation rather than the pressure of original entrapment (Campomenosi et al., 2023a; Mingardi et al., 2023). Notwithstanding the need for careful interpretation, the dependence of elastic thermobarometry on physical properties rather than chemical composition makes it an attractive option in regional studies where the consistency of results obtained from a common rock type, such as orogenic eclogite, enables tectonic hypotheses to be rigorously tested. For example, quartz in garnet barometry on eclogites in the northern Franciscan Complex has been used to falsify a previous interpretation that the eclogite blocks were sourced from different depths in the subduction channel (Cisneros et al., 2022).
Ideally, the equilibrium mineral assemblage records the metamorphic peak at a point on a geotherm crossed during a dynamic evolution from the lower to higher thermal gradient (clockwise P–T–t path) or higher to lower thermal gradient (anti-clockwise P–T–t path). A convenient way to represent the metamorphic peak is the thermobaric ratio, (Brown and Johnson, 2019a), which is more useful than simply T or P alone because there is a natural tendency to highlight extreme values. For example, it has been claimed that the Chasteiran Unit in the Western Alps represents a distinctly colder UHP slice than the Brossasco–Isasca Unit (Manzotti et al., 2022), but in terms of this slice is similar to others that were subducted to higher pressures – the Lago di Cignana site yields of ∼ 185 ∘C GPa−1, the Chasteiran Unit of ∼ 180 ∘C GPa−1 and the Brossasco–Isasca Unit of ∼ 170 ∘C GPa−1 (based on data in Manzotti et al., 2022). Using ± 5 % error for T and P yields an uncertainty in of approximately 10 %. Thus, these three localities appear to have been equally cold.
One interesting feature of some Phanerozoic orogenic belts is the distinctive β-shaped P–T–t paths that have been characterized at multiple localities from the Western Alps to Papua New Guinea (e.g. Kurz et al., 2008; Perraki and Faryad, 2014; Faryad et al., 2019; Bovay et al., 2023). These paths are interpreted to record a two-stage exhumation process, commonly from UHP conditions, as subduction adjusts during collision. Based on numerical modelling (Sizova et al., 2019), the initial close-to-isothermal pressure drop, which is commonly on the order of 1–2 GPa, has been related to slab rollback and vertical extrusion during ongoing continental subduction. In the models, this initial exhumation is followed by a short-lived increase in temperature of up to 200∘, due to conductive heating caused by asthenospheric upwelling, sometimes accompanied by an increase in pressure, before exhumation to Moho depth. In these circumstances, assuming that the cooling rate can be converted to the exhumation rate could be misleading.
In the future we can expect incremental progress in analytical techniques and quantitative methods. Our community will continue to identify new localities that record UHP metamorphism, such as the recent discoveries of coesite in the meta-ophiolitic suite of the Monviso Massif in the Lago Superiore Unit (Ghignone et al., 2023) and in the Eclogitic Micaschist Complex of the Sesia Zone (e.g. Chen et al., 2023), both in the Western Alps. Furthermore, we will continue to refine the P–T conditions and age of metamorphism for known localities, particularly those that have proven to be controversial, such as the eclogites of the northern Belomorian Province of the Fennoscandian Shield (e.g. Melnik et al., 2021; Li et al., 2023). Although determination of P–T conditions is now standard practice in petrological studies, this information still requires careful interpretation, particularly in terms of linking P–T with age. These data will remain an important contribution to future studies because they allow us to evaluate tectonic models and secular change, as discussed below. Much of the work discussed in this section is based on the equilibrium assumption, which has served our community well for more than 40 years. However, it is likely that further progress in understanding metamorphic and orogenic processes will require the application of thermodynamics under nonhydrostatic stress conditions (e.g. Zhong et al., 2017; Powell et al., 2018; Wheeler, 2020; Cionoiu et al., 2022).
In general, we should anticipate only incremental improvements to thermodynamic datasets (e.g. Holland et al., 2022) and single-element thermobarometers (e.g. Kohn, 2020; Osborne et al., 2022), although we can expect another generation of activity–composition models, likely with improvements to Fe3+ partitioning and the solubility of species in fluids and melt as a function of P–T–X, where X is the bulk chemical composition of the equilibration volume. Cases such as the study of serpentinite-hosted alloys will require new thermodynamic data and activity–composition models for many of the alloys of interest (Evans et al., 2023). By contrast, elastic barometry is a fast-developing method with scope for much wider use in the future and ongoing expansion of the range of minerals to which the method can be applied (e.g. Gonzalez et al., 2021; Ehlers et al., 2022). We will continue to develop new approaches and tools for modelling, such as the recent approach in which thermodynamic modelling has been linked with modelling oxygen isotope fractionation in open systems with contrasting lithologies (Vho et al., 2020). This integrated approach allows us to track fluid migration, to distinguish channelized from porous flow, and to determine the P–T conditions and extent of fluid–rock interaction during subduction. Furthermore, our community will continue to develop methodologies to deal with rocks that have been partially overprinted during exhumation or due to polymetamorphism.
Lastly, we should expect that we will resolve differences between disparate datasets, such as the controversy over whether rocks record peak metamorphic conditions that are inconsistent with (i.e. hotter than) models of the temperature profiles along contemporary slab tops (e.g. Penniston-Dorland et al., 2015; van Keken et al., 2018; Brown and Johnson, 2019b). For example, Agard et al. (2018) used a dataset of 130 samples retrieved from within 500 m of the plate interface to show that typical prograde P–T paths for subduction interface markers in numerical models may not be significantly cooler than the natural data. Furthermore, recent modelling studies suggest that subduction-related metamorphic rocks may not record conditions connected to steady-state continuous subduction but rather may record conditions either linked to the early or the late stages of a subduction cycle (Wang et al., 2023), as suggested earlier by Agard et al. (2009), or related to subduction of young ocean lithosphere (Kerswell et al., 2023).
2.2 From geochronology to petrochronology
The history of geochronology is comprehensively covered in a plethora of textbooks and short course volumes (e.g. Hanchar and Hoskin, 2003). For the purposes of this review, it is sufficient to emphasize that the most significant advance in relation to studies of eclogites has been the advent of in situ analytical techniques, as summarized in Kohn et al. (2017). Here, I briefly summarize the two main advances related to studies of eclogites. First, beginning in the late 1990s, was the application of SIMS (secondary ion mass spectrometry) dating to zircon in eclogites, including studies by Brueckner et al. (1998) in the Northeast Greenland Eclogite Province, Rubatto et al. (1998, 1999) in the Zermatt–Saas Fee ophiolites and in the Sesia–Lanzo Zone, and Hacker et al. (1998) in the Qinling–Dabie orogen. Second, during the 2000s, came the introduction of LA-ICP-MS (laser ablation inductively coupled plasma mass spectrometry) followed by split-stream dual LA-ICP-MS, which increased access to geochronology and led to the development of petrochronology (e.g. Kohn et al., 2017), making age determinations an essential and routine part of any study of eclogites.
Geochronology and petrochronology are disciplines in which there are continual advances, such as the recent developments in microsampling. For a mineral such as garnet, which grows over a P–T interval during a period of time to form porphyroblasts, this advance allows for analysis of individual growth zones that have different compositions or textures to address rates of mineral growth and rates of tectonic processes. Furthermore, microsampling allows for analysis of matrix garnet grains in rocks without porphyroblasts (e.g. Tual et al., 2022). Moreover, an existing technique can be extended to a new mineral suite, for example, the successful demonstration that U–Pb geochronology of metamorphic perovskite can be used to date deformation and fluid flow in subducted ocean lithosphere (Piccoli et al., 2023). Lastly, new technologies are being developed and introduced both to increase the range of elemental isotopes that can be analysed and to improve the spatial resolution of analyses. In particular, the use of a hexapole collision/reaction cell to remove mass interferences in laser ablation tandem inductively coupled plasma mass spectrometry (LA-ICP-MS/MS or LA-ICP-QQQ, triple quadruple) allows for rapid in situ determination of isotope ratios in a variety of minerals (Simpson et al., 2021). This method has been shown to be useful in campaign style in situ Lu–Hf dating of garnet from eclogite facies rocks in the HP–UHP Western Gneiss Region of Norway, where polymetamorphism in some localities was recognized for the first time (Tamblyn et al., 2022b), and in a study of garnets from the Moine Supergroup of northern Scotland, a well-known polymetamorphic terrane (Simpson et al., 2023).
2.3 Cooling and exhumation – size and age matter
We can use geochronology (at higher temperatures) and thermochronology (at lower temperatures) on minerals with different closure temperatures to determine cooling and/or exhumation rates. Alternatively, we can combine peak P–T with time information obtained by inverting concentration gradients created by elemental diffusion in minerals such as garnet during cooling and exhumation (diffusion chronometry). Diffusion chronometry is generally limited to the high-temperature segment of the post-peak evolution. Exhumation rates are commonly calculated from time-averaged cooling rates using the lithostatic approximation (P=ρgz, where ρ is the density, g is the gravitational acceleration and z is depth). Taking errors associated with both pressure and cooling rate into account, the uncertainties in exhumation rates can be close to 100 %, for example, as modelled for the central Sulu Belt (Wang et al., 2023).
Cooling and exhumation rates retrieved from HP–UHP localities based on geochronology can vary by an order of magnitude, e.g. from several millimetres per year to several centimetres per year, as recorded at different localities in the Western Alps (Manzotti et al., 2018). Furthermore, rates of cooling and exhumation of HP–UHP rocks determined using diffusion chronometry can be about an order of magnitude faster again (tens of centimetres per year) than the fastest rates determined using geochronology, for example, as determined from zoning in garnet in the Tianshan of northwestern China (Schwarzenbach et al., 2021) and the Ottawan phase of the long-lived Grenville orogeny (Fan et al., 2023). This difference in rates between those based on geochronology and those based on diffusion chronometry is a general observation for all types of metamorphism (Chowdhury et al., 2021; Brown et al., 2022; Zou et al., 2023), with results from diffusion chronometry interpreted to record atypical and transient thermal conditions during short-duration metamorphism rather than the ambient thermal conditions for the tectonic setting in which the rocks formed (Viete and Lister, 2017; Fan et al., 2023). Fast rates of exhumation that exceed tectonic rates of deformation require an alternative buoyancy-driven mechanism of exhumation, such as channel or diapiric flow (Hacker and Gerya, 2013). Although exhumation from high-pressure conditions is quite well understood in general (Hacker and Gerya, 2013), the exhumation rate of crustal rocks from HP–UHP conditions, using rates based on a pressure-to-depth conversion assuming lithostatic pressure, appears to vary with the size of the unit being exhumed, with smaller HP–UHP slices being exhumed at plate tectonic rates and larger HP–UHP terranes being exhumed at slower rates (Kylander-Clark et al., 2012; Hermann and Rubatto, 2014). However, the size of individual HP–UHP units and the UHP component is not well known in many cases, and the volume of continental crust subducted to (and returned from) ultrahigh pressures and the proportion lost to the mantle are largely unknown.
At one end of the spectrum are the UHP domains in the Western Alps, which are small and represent an almost negligible proportion of the subducted continental crust. Classic examples include the Brossasco–Isasca Unit in the Dora Maira Massif, which is a tectonic sliver on the order of 10 km in length and 1 km in thickness (Castelli et al., 2007), in which UHP mineral assemblages are generally limited to lenses of pyrope quartzite and marble from metres to tens of metres in length within country rock gneisses and schists (Schertl et al., 1991; Castelli et al., 2007), and the Lago di Cignana Unit, which comprises a sequence of tectonically dismembered boudins from 100 m to > 1 km long and < 150 m thick (Forster et al., 2004). More recently, Luisier et al. (2019) have described pipes 10–50 m across with “whiteschist” mineral assemblages, inferred to represent hydrothermally altered zones within metagranite in the Monte Rosa nappe, and Manzotti et al. (2022) have reported the discovery of a new UHP unit in the northern Dora Maira Massif that is only a few tens of metres thick.
At the other extreme are orogenic belts where tectonic units are inferred to be regional in scale, with UHP metamorphic domains that are kilometres thick and cover thousands of square kilometres and represent a substantial proportion of the subducted continental crust. For example, the UHP zone of the Sulu Belt in eastern China comprises at least four tectonic slices, each several kilometres thick, within which UHP metamorphism has been confirmed based on coesite-bearing domains in zircon with ages from 235 to 225 Ma from both eclogites and their host gneisses (Xu et al., 2009; Liu and Liou, 2011). Also, in the Western Gneiss Region in Norway, Spengler et al. (2021) and Spengler and Alifirova (2022) have used P–T data from widely distributed eclogite and peridotite bodies in felsic gneisses to show that UHP metamorphic rocks occur over a much larger area than previously inferred, now approximately 10 000 km2, stretching along the coast from Nordfjorden to Moldefjorden and up to 100 km into the hinterland.
One reason for the meager amount of data is that identification of HP–UHP terranes commonly relies on quantitative data from mafic (and ultramafic) enclaves enclosed within strongly foliated felsic gneisses that generally lack diagnostic HP–UHP minerals and, therefore, cannot be shown to be cofacial (e.g. Young and Kylander-Clark, 2015). However, Schorn (2022) has shown that there are differences in the prograde devolatilization of mafic and felsic rocks during subduction, with devolatilization of mafic rocks being sufficient to maintain high reaction rates that allow for growth of the peak mineral assemblage, whereas the limited intrinsic H2O content of felsic rocks can lead to fluid-absent conditions that would impede equilibration at the outcrop scale. Furthermore, in the felsic rocks the reaction products are likely to form along grain boundaries and will be vulnerable to obliteration by deformation during exhumation, removing evidence of UHP conditions (Schorn, 2022). These differences would allow for eclogites and their host gneisses to have been subducted and exhumed together, without the latter necessarily remembering the journey.
Although mapping the areal distribution of HP–UHP rocks and quantifying the rate of exhumation in any orogenic belt are formidable tasks, these data are primary information required to evaluate exhumation mechanisms. Given the limited number of examples to date, uncertainty over the volume of crust subducted to mantle conditions and the wide variation in the rates of exhumation, it is unclear what, if anything, these data tell us about exhumation mechanisms. Furthermore, whether the faster rates are an artefact of heterogeneous tectonic pressure preferentially affecting smaller lithologically distinct domains is unresolved, and, thus, whether the rates are truly bimodal is uncertain.
We have long known that cooling rates, and by implication exhumation rates, of metamorphic rocks were faster during the Phanerozoic than during the Precambrian (Dunlap, 2000; Willigers et al., 2002). Secular change in metamorphic cooling rates has been studied in more detail recently by Scibiorski et al. (2015), Chowdhury et al. (2021) and Brown et al. (2022), using successively larger datasets, and Zou et al. (2023), who also explicitly included exhumation rates. The secular trend that emerges from these studies reveals faster cooling/exhumation rates in the Phanerozoic than in the mid-Paleoproterozoic, with a low from the late Paleoproterozoic to the early Neoproterozoic (Zou et al., 2023) that coincides with a dearth of low- metamorphic localities in Mesoproterozoic orogens (Brown et al., 2022). The mid-Proterozoic low is associated with the Columbia and Rodinia supercontinents, where continental insulation (Tamblyn et al., 2022a; Zou et al., 2023) and a plate slowdown (Sobolev and Brown, 2019; O'Neill et al., 2022) reduce the rate of secular mantle cooling leading to a warmer style of orogenesis (Spencer et al., 2021).
Many of the data used in the studies quoted above use time-averaged rates of cooling and exhumation, which are generally insufficient to characterize or understand complex, nonsteady exhumation processes (e.g. Smye et al., 2018). To differentiate among different exhumation mechanisms for eclogites will require more detailed studies of the rates of cooling and exhumation, using the full range of chronometric methods available to us. To accomplish this goal, we will need to continue to improve in situ analytical techniques and diffusion modelling and apply these methods more widely in studies of eclogites. Moreover, we need to develop a better understanding of the underlying tectonothermal processes controlling differences in cooling/exhumation rates between those determined using geochronology and those based on diffusion chronometry.
2.4 Use of detrital materials
The development of petrochronology provided the tools to study millimetre size eclogite clasts in a synorogenic basin in the northern Canadian Cordillera, allowing for determination of P–T and using rutile thermochronology and deposition age, average cooling and exhumation rates (Kellet et al., 2018). Also, following the use of detrital garnet composition to characterize sediment sources in provenance studies, mineral inclusions in detrital garnets, e.g. identified using Raman spectroscopy, are now being used to reveal exciting new details about the record of HP–UHP metamorphism (Schönig et al., 2022). For example, for two proximal modern sand samples from the HP–UHP sector of the Western Gneiss Region in Norway, the compositions and mineral inclusion suites for about 300 detrital garnets indicate derivation from catchments including upper amphibolite, HP granulite, and eclogite facies rocks with both mafic and felsic protoliths (Schönig et al., 2018a, b). Furthermore, coesite inclusions were discovered in some of the detrital garnets. More spectacularly, in a suite of several hundred inclusion-bearing detrital garnets retrieved from sand in streams draining the UHP metamorphic zone around the Saidenbach Reservoir in the central Erzgebirge (northwestern Bohemian Massif, Germany), more than two dozen garnets from five samples yielded over 40 mainly monomineralic coesite inclusions and more than 20 garnets from a sixth sample contained some 40 diamond inclusions (Schönig et al., 2019). Also, coesite has been discovered as inclusions in detrital garnets from a modern placer deposit in the Late Miocene to recent HP–UHP metamorphic terrane of eastern Papua New Guinea (Baldwin et al., 2021). In this case, the composition of the coesite-bearing detrital garnets indicate they were sourced from felsic protoliths, which is significant because the only in situ coesite grain found previously was from an eclogite. The Baldwin et al. (2022) study emphasizes the importance of tracing UHP metamorphism at the catchment scale rather than being limited to blocks of eclogite in country rock. Importantly, the sedimentary record can archive mineralogical evidence of UHP metamorphism from felsic basement rocks (Schönig et al., 2021), where the mineralogical evidence for a UHP history has long been ambiguous (Young and Kylander-Clark, 2015; Schorn, 2022), which opens up new opportunities for the prospective exploration of UHP metamorphism and its first appearance in the geological record.
The massive increase in computing power since 1965, when Gordon Moore – cofounder of Intel – postulated that the number of transistors that can be packed into a given unit of space will double every 2 years, has enabled corresponding advances in the use of numerical modelling to understand subduction zone processes. Early numerical models of subduction tectonics and exhumation of HP metamorphic rocks include those by Gerya et al. (2002) and Jolivet et al. (2003). These were followed by studies addressing whether subduction and plate tectonics, as well as the formation and exhumation of HP and UHP metamorphic rocks, were plausible during the Precambrian (O'Neill et al., 2007; van Hunen and van den Berg, 2008; Sizova et al., 2010, 2012, 2014; Perchuk et al., 2019; Chowdhury et al., 2020). In parallel, Hacker et al. (2003a) linked petrological modelling with models of subduction zone tectonics, which led to models that provided insight into the mechanisms of fluid generation and egress during subduction (Hacker et al., 2003b; van Keken et al., 2011) and, importantly, the relationship between hydrate breakdown reactions in the blueschist facies and intermediate-depth earthquakes (Abers et al., 2013). The power of the modelling approach has been emphasized recently by a comprehensive study of metamorphism and deformation along the subduction interface (Smye and England, 2023) and by a study of the eclogitization of dry and impermeable granulite by fluid flow with reaction-induced porosity (Bras et al., 2023). In some circumstances a numerical modelling approach may be the best way forward (Stern and Gerya, 2018; Lu et al., 2021), such as by investigating the difficult problem of how subduction can be initiated particularly in circumstances where preexisting weaknesses, such as transform faults, were absent, as might have been the case if plate tectonics evolved from a precursor tectonic mode in the Archean. For example, could subduction in the Archean (or Hadean) have been initiated by endogenic processes such as mantle plumes (Gerya et al., 2015; Baes et al., 2020, 2021) or exogenic processes such as impacts (O'Neill et al., 2017, 2020)? As computers reach the physical limits of Moore's law, probably during this decade, we are about to enter the age of quantum computing, which undoubtedly will lead to new breakthroughs in modelling the evolution of eclogites and related rocks in relation to subduction zone processes and collisional orogenesis. For example, we can expect that petrological–thermomechanical numerical models will incorporate more sophisticated thermodynamic modelling, as demonstrated by Smye and England (2023), and we can expect to resolve differences between records retrieved from rocks and those generated in models, as discussed at the end of Sect. 2.1.
Finding evidence of eclogites in the geological record has important implications for understanding Earth's geodynamic evolution. Since the Archean, orogenic eclogites are generally related to subduction (Brown and Johnson, 2019b). Therefore, orogenic eclogites appearing abundantly in the geological record may identify the widespread stabilization of subduction on Earth (Sobolev and Brown, 2019). By contrast, before the Proterozoic, xenolithic eclogites are related to both subduction of oceanic lithosphere (Aulbach and Smart, 2023) and delamination of the lower crust (Johnson et al., 2014). Lastly, abundant evidence of UHP metamorphism in the geological record since the Proterozoic likely identifies the widespread occurrence of deep subduction of continental lithosphere, perhaps related to secular cooling and deeper slab breakoff (Sizova et al., 2014).
4.1 Eclogites and secular change in metamorphism
Metamorphism of crustal rocks has occurred since the Eoarchean, but there are few studies of metamorphic rocks older than Mesoarchean. To address secular change in metamorphism, Brown (2007) compiled a dataset of 144 metamorphic P, T and t determinations and grouped them into three kinds of metamorphism by rock type, as follows: granulite–ultrahigh-temperature metamorphism, medium-temperature eclogite–high-pressure granulite metamorphism and high-pressure–ultrahigh-pressure metamorphism. With time this dataset has grown to 567 localities from the Cenozoic to the Eoarchean with a wider variety of rock types and three kinds of metamorphism have become defined in terms of their range of values, as follows: low- metamorphism ( < 375 ∘C GPa−1), intermediate- metamorphism ( 375–775 ∘C GPa−1) and high- metamorphism ( > 775 ∘C GPa−1) (Brown and Johnson, 2018, 2019a, b).
These data have been used to investigate the spatiotemporal distribution of metamorphism (Liu et al., 2022) and secular change (Holder et al., 2019; Brown et al., 2020, 2022) but not without controversy in relation to the scarcity of low- metamorphism, characterized by blueschists and/or eclogites, prior to ca. 700 Ma (Fig. 1). The spread of ratios recorded by the crust increased from ca. 2.8 Ga to a maximum at ca. 1.8 Ga, before narrowing slightly along a trend to lower values (Holder et al., 2019, their Fig. 3). Within this overall pattern, eclogite occurs in a small number of localities in the Paleoproterozoic and Mesoproterozoic (Fig. 1), associated with the amalgamation of the supercontinents Columbia and Rodinia, before the widespread appearance of blueschists and eclogites, generally with values below 600 ∘C GPa−1, since ca. 700 Ma (Fig. 1), in locations concentrated in Eurasia rather than the Gondwanan continents (Liu et al., 2022).
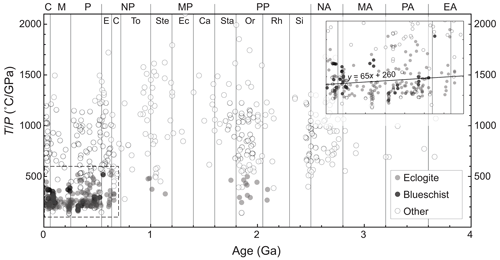
Figure 1Metamorphic thermobaric ratio () for 578 localities distributed worldwide plotted against age (dataset with minor corrections from Brown and Johnson, 2019c). Eclogites and blueschists are emphasized with shading, but other rock types are not distinguished. Note the absence of blueschists before the late Cryogenian and the scarcity of eclogites before the late Tonian. The inset emphasizes eclogites and blueschists younger than 800 Ma, and the linear regression through these data demonstrates a secular decrease in during this interval. The abbreviations in the top row are C – Cenozoic, M – Mesozoic, P – Paleozoic, NP – Neoproterozoic, MP – Mesoproterozoic, PP – Paleoproterozoic, NA – Neoarchean, MA – Mesoarchean, PA – Paleoarchean and EA – Eoarchean. The abbreviations in the second row are E – Ediacaran, C – Cryogenian, To – Tonian, Ste – Stenian, Ec – Ectasian, Ca – Calymmian, Sta – Statherian, Or – Orosirian, Rh – Rhyacian and Si – Siderian.
The paucity of eclogites from the mid-Paleoproterozoic essentially until the late Neoproterozoic coincides with the period of low cooling and exhumation rates discussed earlier and is also related to the warmer style of orogenesis associated with the tenure of the supercontinents Columbia and Rodinia (Spencer et al., 2021) and the resulting warmer subcontinental mantle due to continental insulation (Tamblyn et al., 2022a; Zou et al., 2023) and an associated plate slowdown (Sobolev and Brown, 2019; O'Neill et al., 2022). The absence of blueschists prior to the late Neoproterozoic is enigmatic. Although secular change in protolith composition has been speculated as the cause of this absence (Palin and White, 2016), in fact the low-MgO basalt compositions required for glaucophane-bearing mineral assemblages to develop (≤ 11.2 wt % MgO) have been widely available in greenstone belts since the Paleoarchean (Condie et al., 2016) and occur, for example, as members of block in matrix ophiolitic melanges along an inferred arc–passive continental margin suture formed at ca. 2.5 Ga in the North China Craton (Wang et al., 2019; Jiang et al., 2020).
In investigating secular change, it is critical to assess whether the temporal distribution of metamorphic rocks is broadly representative or is biased to the point of being unreliable. In addition to the possibility of sampling bias related to the tendency to concentrate on spectacular localities, there are two issues to consider: preservation – bias in the rock record due to a difference between what was created (due to burial) and what was preserved (due to exhumation) – and survivorship – further bias due to differences between what was originally preserved and what has been lost by erosion. Maybe the scarcity of orogenic eclogites in the Proterozoic and their absence from the Archean record is due to these biases. However, there is no a priori reason why exhumation would have been less effective in the Precambrian, since the depth of erosion of orogens varies throughout the Proterozoic and Phanerozoic (Weller et al., 2022). More likely, as discussed below, a warmer mid-Proterozoic mantle and style of orogenesis could have precluded the widespread formation of eclogites (Spencer et al., 2021; Tamblyn et al., 2022a).
In the case of orogenic eclogites, one reason why preservation should not be a first-order problem is that buoyant felsic continental crust, with which eclogites are commonly associated as boudinaged relics, should resist recycling into the mantle (Wang et al., 2021). Thus, if an appropriate tectonic setting with a low thermal gradient was available, basaltic rocks emplaced along continental margins during extension, breakup and passive margin formation should transform to eclogite during subsequent subduction and collision and should be preserved in the orogenic crust. Furthermore, because older orogens do not necessarily expose deeper crust (Weller et al., 2022), some of the preserved eclogites should survive rather than all being lost to erosion. These considerations, coupled with the absence of orogenic eclogites from the Archean rock record, suggest that an appropriate tectonic setting with the potential for preservation was not available (Sizova et al., 2014; Chowdhury et al., 2020).
Accepting that mantle potential temperature (TP) was higher in the past (Herzberg, 2022a, b; Herzberg et al., 2010), it is important to know whether subduction prior to the Cryogenian could have generated low thermal gradients that are now not represented in the surviving record of metamorphism. The answer is ambiguous for multiple reasons. In numerical experiments of intraoceanic subduction and continental collision at a mantle of TP > 100 ∘C warmer than the present day, slab dynamics are different with large-scale trench rollback and slab breakoff at depths that generally preclude the formation of low- rocks (Perchuk et al., 2019; Sizova et al., 2014). By contrast, Precambrian eclogites preserved in the cratonic lithospheric mantle record of 180–220 ∘C GPa−1, similar to Phanerozoic blueschists and orogenic eclogites (Aulbach and Smart, 2023). However, although the mechanisms by which the cratonic lithospheric mantle was formed and the cratonic eclogites were preserved are both uncertain, the P–T conditions (3–6 GPa, 700–1250 ∘C) locate these eclogites at depths above the lithosphere–asthenosphere boundary (Aulbach and Smart, 2023). Thus, the low range (180–250 ∘C GPa−1) likely records the conditions during craton formation rather than subduction.
It is possible that hotter mantle geotherms (Herzberg, 2022a, b; Herzberg et al., 2010) combined with slower average metamorphic cooling rates (Chowdhury et al., 2021; Brown et al., 2022; Zou et al., 2023) and a different tectonic style (Sizova et al., 2014; Chowdhury et al., 2020) earlier in Earth history could have led to more widespread overprinting of eclogites during exhumation, whereas cooler mantle geotherms and faster average cooling rates since the Mesoproterozoic (Zou et al., 2023) could have limited retrogression of such rocks. However, as was pointed out a long time ago by Coleman et al. (1965), there are “eclogites and eclogites”. Overprinting is common in eclogites characterized by higher values (“Group B” eclogites of Coleman et al., 1965), which are commonly associated with high-pressure granulites and migmatites (with characteristic of intermediate- metamorphism), as occur throughout the Proterozoic and Phanerozoic rock record (Wang et al., 2021). The overprinting may be related to a combination of slower cooling rates and longer exhumation times for these eclogites (Wang et al., 2021). By contrast, overprinting is less common in eclogites characterized by lower values (“Group C” eclogites of Coleman et al., 1965), which are characterized by faster cooling rates and shorter exhumation times (Wang et al., 2021). In a young orogenic system, such as the Himalayas, this contrast in eclogite types may be related to their generation during different stages of the orogenic cycle, viz. after significant crustal thickening by underplating and during initial collision, respectively (Wang et al., 2021). That eclogites with low values are largely absent from the record during the mid-Proterozoic, a time when mountain belts tended to be hotter and thinner, with lower orogenic relief, due to a warmer mantle (Spencer et al., 2021; Zou et al., 2023), should not be a surprise. Notwithstanding, overprinting, which is a common feature of Group B eclogites, should not generate bias through time.
UHP metamorphic rocks are variably distributed but occur throughout the geological record since ca. 700 Ma with no protracted gaps (Brown and Johnson, 2019a, b, c). By contrast, before ca. 700 Ma, UHP metamorphic rocks are effectively absent from the rock record, except for four occurrences in the Orosirian (2050–1800 Ma). If we accept the lithostatic assumption, subduction of continental lithosphere to mantle depths requires that collision slab breakoff occurs after the continental edge has reached UHP conditions at a depth below the continental edge; otherwise the lithosphere is recycled back into the mantle (Sizova et al., 2014). It appears that this condition has been met since ca. 700 Ma but only locally in the mid-Paleoproterozoic before ca. 700 Ma. Consistent with this interpretation, there is no evidence from the mantle isotope record of recycled upper continental crust before the Phanerozoic (Doucet et al., 2020; Jackson and Macdonald, 2022), which suggests that shallow slab breakoff or a change in subduction dynamics may have been the limiting factor in the secular distribution of eclogites with low values (Sizova et al., 2014; Chowdhury et al., 2021).
4.2 Eclogite metamorphism and plate tectonics
Plate tectonics is a mode in which plates have generally strong interiors but are separated by weak boundaries. A theory of mantle convection that generates plates remains elusive and is one of the grand challenges of geodynamics. Regardless, the null hypothesis for Earth's global tectonic mode should be that of plate tectonics back to the Hadean. In this context, it would be useful to know how far back in time evidence of plate like behaviour can be traced in the geologic record, since this would provide a minimum age for the emergence of (global) plate tectonics. Unfortunately, there is no consensus interpretation of the available data.
Whether limited occurrences of approximately coeval eclogites in the Proterozoic are a record of (global) plate tectonics or simply of regional subduction and collision is debatable, but individual occurrences of eclogites isolated in time at best can only be a record of local subduction. However, based on a global dataset, the spatiotemporal distribution of metamorphism is not uniform through time, but rather regional metamorphism occurred in age clusters at > 2.2, 2.2–1.4, 1.4–0.85, 0.85–0.2 and < 0.2 Ga that are clearly related to the supercontinent cycle (Brown and Johnson, 2018; Holder et al., 2019; Liu et al., 2022). Furthermore, Holder et al. (2019) have shown that metamorphism during the last 0.2 Ga is characterized by a bimodal distribution of and that this bimodality developed progressively during the Paleoproterozoic, consistent with an evolving plate tectonic mode. Consistent with this conclusion, Zhao et al. (2022) have shown that fossil subduction zones, as inferred from seismic studies, had shallower dips and shorter lengths in the Proterozoic when compared to those in the Phanerozoic. This may reflect a change in the depth of slab breakoff due to mantle cooling, as modelled by Sizova et al. (2014).
Based on this record, what can we learn about the evolution of plate tectonics since the Archean? Using nonparametric statistics, we can search for statistically significant change points in time series data, such as metamorphic or cooling rate or mantle TP (Brown et al., 2020, 2022). A change point identifies a minimum age for a state shift, i.e. crossing a threshold to a new stable state. There are change points in the metamorphic data in the Paleoproterozoic, which have been interpreted to record a state shift to more efficient cooling of the mantle (Brown et al., 2020, 2022), consistent with stable subduction and amalgamation of the megacontinent of Nuna, the core of the supercontinent Columbia, by plate tectonics processes (Wan et al., 2020). Change points in the metamorphic data in the Paleozoic have been interpreted to record a state shift to lower thermal gradients along the subduction interface and subduction of continental margins to UHP metamorphic conditions (Brown et al., 2020, 2022), consistent with deeper slab breakoff (Sizova et al., 2014). These changes may relate to lubrication of subduction by an increased sediment supply at continental margins and in trenches after the major surface erosion events in the Paleoproterozoic, the Huronian glaciations from 2.45 to 2.2 Ga, leading to the formation of the Columbia supercontinent and, in the Neoproterozoic, the “snowball” Earth glaciations from 0.75 to 0.63 Ga, leading to the formation of the Pangaea supercontinent (Sobolev and Brown, 2019). The mid-Proterozoic from about 1.75 to 0.75 Ga was likely a period of delayed mantle cooling due to continental insulation and reduced plate tectonic activity, which may explain the scarcity of eclogites during this interval (Tamblyn et al., 2022a; O'Neill et al., 2022; Zou et al., 2023).
4.3 Diamonds
In this section, I briefly summarize information retrieved from minerals included in diamonds formed in and returned from the continental lithosphere and the deeper mantle. These diamonds, whose petrogenesis is clearly distinct from the metamorphic (noncratonic) diamonds discussed earlier, are sampled and transported by kimberlite magmas during their ascent to the surface.
It has been more than a decade since Shirey and Richardson (2011) demonstrated a change in inclusion mineralogy in diamonds from the Kaapvaal Craton, one of the oldest stabilized cratons, from solely a peridotitic mineral assemblage (chromium-rich pyrope, diopside, enstatite, chromite or olivine) before 3.0 Ga to both peridotitic and eclogitic (pyrope–almandine, omphacite or coesite) mineral assemblages after 3.0 Ga. These authors interpreted the change in mineralogy to record stable subduction as early as the Mesoarchean associated with final amalgamation of this craton. Subsequent periods of diamond growth in both the Kaapvaal and Zimbabwe cratons correlate with orogenic events broadly related to the supercraton–supercontinent cycle and/or well-documented magmatic episodes (Smit et al., 2022). The limited age data available for inclusions in lithospheric diamonds from other cratons suggest a transition to eclogitic mineral assemblages in the Paleoproterozoic (Smit et al., 2022). Although speculative, this range of ages may record a diachronous transition from a precursor tectonic mode to plate tectonics.
Importantly, there is now a growing number of studies of superdeep (sublithospheric) diamonds that appear to have come from the top of the lower mantle, based on mineral inclusion suites (including former bridgemanite, ferropericlase and a calcium ferrite phase). In one case, ferropericlase inclusions have been used to estimate minimum P–T conditions of ∼ 16 GPa and ∼ 1550 ∘C, interpreted to record the point during the return from the lower mantle at which plastic deformation of the diamond ceased (Alvaro et al., 2022). Measurement of the elemental and isotope composition of these inclusions should lead to a better understanding of how the deep interior and surface are linked in the context of Earth as a fully coupled system.
Although age information for superdeep diamonds is generally lacking, they are probably Mesoproterozoic or younger and, based on the age of the transporting kimberlites, range from ca. 1.15 Ga to ca. 90 Ma (Smith et al., 2018; Smit et al., 2022). A single example of a directly dated CaTiSi perovskite inclusion in diamond from the Juína area in Brazil yielded a 206Pb–238U age of 101 ± 7 Ma, within the uncertainty in the age of the kimberlite eruption at 93.1 ± 1.5 Ma (Bulanova et al., 2010). Diamond formation at Juína was probably related to subduction of Mesozoic age ocean lithosphere into the lower mantle (Harte and Richardson, 2012). These data raise the intriguing possibility that deep subduction of ocean plates into the lower mantle might be a late Mesoproterozoic to Cenozoic phenomenon. If this interpretation is correct, it presages the ability of a subducting ocean slab to drag continental lithosphere to mantle depths beginning in the late Cryogenian (Sizova et al., 2014; Brown et al., 2022). Clearly, there is an urgent need for more data from superdeep diamonds because these are the archive of secular change in the deep mantle. In particular, and notwithstanding the difficulty, we should test speculation about differences in the ages of lithospheric and sublithospheric diamonds by dating inclusions from as many localities as possible.
Stress in the crust is anisotropic, and the resulting tectonic pressure (a.k.a. “overpressure”) may explain the growth of high-pressure minerals without the need for burial to great depth (e.g. Rutland, 1965; Mancktelow, 1995, 2008; Berg and Gerya, 2005; Pleuger and Podladchikov, 2014; Gerya, 2015; Simon et al., 2023). At the grain scale, variation in pressure, whether due to the physical properties of different minerals in a polycrystalline rock or volume increase during metamorphic reaction, has implications for thermodynamic equilibrium, the interpretation of microstructures and the deformation of metamorphic rocks (e.g. Moulas et al., 2013; Tajčmanová et al., 2014, 2015; Plümper et al., 2022). Furthermore, variation in stress is required to explain many deformation structures from outcrop to the crustal scale, especially where these involve pressure-dependent fracture, pressure solution, or migration of fluids or melts (e.g. Petrini and Podladchikov, 2000; Connolly and Podladchikov, 2004; Mancktelow, 1995, 2008; Brown et al., 1995; Brown, 2010; Moulas et al., 2022; Muñoz-Montecinos et al., 2023). In this context, we may think of metamorphic pressure at a point in the crust as being close to mean stress, which need not be close to the lithostatic load (Moulas et al., 2019). Furthermore, since variations in mean stress can occur at constant depth, variations in metamorphic pressure and hence, possibly, mineral assemblage should be expected to occur at similar structural depth in the crust (e.g. Luisier et al., 2019, 2023). From this perspective, we should consider whether a single occurrence, or even a few occurrences, of a UHP metamorphic indicator mineral, such as coesite, requires a particular tectonometamorphic rock unit to have been subducted to UHP conditions if similar pressures are not generally recorded throughout in the unit. For example, could the newly discovered occurrence of coesite in the Sesia Zone of the Western Alps (Chen et al., 2023) be related to regional variation in mean stress? The coesite is present as inclusions in garnet from a jadeite-bearing leucocratic orthogneiss layer that occurs within gneisses and is exposed in the Argentera quarry near Settimo Vittone in the lower Aosta Valley on the southeastern side of the Eclogitic Micaschist Complex. Multiple studies along the length of the Eclogitic Micaschist Complex during the past 30 years, as summarized by Vho et al. (2020; see also Regis et al., 2014; Giuntoli et al., 2018), have shown that the complex generally records maximum pressures of ∼ 2.0 GPa at 550–650 ∘C. This generalization is confirmed by new results from jadeite-bearing samples collected from a quarry near Tavagnasco, about 2.5 km west-southwest of the Argentera quarry, where phase equilibrium modelling for two samples from the same orthogneiss layer as that studied by Chen et al. (2023) yielded P–T conditions of 550±50 ∘C and 18±2 kbar (Gilotti et al., 2023). Plausibly the occurrence of coesite could be related to tectonic pressure, which becomes significant at P ∼ 2.0 GPa (Yamato et al., 2007; Gerya, 2015). Accepting that variations in pressure must occur, is there evidence for variations in tectonic pressure at the orogen scale?
Consider the structural evolution of the Adula nappe, part of the Penninic nappe stack on the eastern flank of the Lepontine structural and metamorphic dome in the Central Alps. Here, to determine whether the abnormally high pressures recorded were due to a component of tectonic overpressure, Pleuger and Podladchikov (2014) made a purely structural kinematic restoration of the structural architecture. The resulting restoration allows for an estimate to be made of the depth of burial of the Adula nappe and the associated Penninic units, without using metamorphic pressures to pin the nappes to a particular depth at a given time based on the lithostatic approximation. For the maximum burial stage of the Adula nappe, estimates of local tectonic pressure are 1.4 to 1.8 times the maximum lithostatic pressure (Plith), whereas those for the overlying Tambo nappe are broadly in agreement with Plith. If tectonic overpressure is accepted as an explanation, the Adula nappe was probably not subducted to the mantle depth. Assuming the structural record of the Penninic nappe stack is complete, decay of tectonic pressure may be a plausible explanation for decompression from eclogite to amphibolite facies conditions during thrusting (Pleuger and Podladchikov, 2014).
Similarly, based on a study of compositional zoning in garnet from eclogites in the Western Gneiss Region of Norway, Simon et al. (2023) proposed isothermal compression from 1.1–1.6 GPa at 510–600 ∘C to a pressure peak of 2.8 GPa at 600 ∘C, followed by near-isobaric heating to 660–680 ∘C, before near-isothermal decompression of more than 1.4 GPa, based on P–T determined from fine-grained clinopyroxene–amphibole–plagioclase symplectites around omphacite. These authors argued that such an evolution can be explained if ∼ 1.4 GPa of tectonic pressure was imposed as the eclogites crystallized at constant depth and temperature during the collisional stage of the orogeny, followed by the formation of the symplectites during decay of the tectonic pressure.
Are there artefacts of tectonic overpressure recorded in other orogens that have not been recognized? Based on metamorphic P–T and age data for a suite of HP amphibolite and granulite samples together with a single eclogite sample from the Dunhuang area in northwestern China, Wang et al. (2022) argued for a change in the thermal gradient of subduction at ca. 420 Ma, with subduction becoming colder due to a plate reorganization and slab rollback. However, a review of the data suggests that tectonic overpressure could provide an alternative explanation for the observed change that is equally likely. Although the samples span an age range from ca. 460 to ca. 410 Ma, they fall into three age groups, as follows. The first group contains five samples older than 420 Ma, with ages from 463 ± 13 to 430 ± 6 Ma and similar P–T conditions of 1.35–1.5 GPa (mean of 1.44 ± 0.12 GPa, 2 SD) at 730–843 ∘C (mean of 797 ± 92 ∘C, 2 SD) with no clear temporal trend (Wang et al., 2022, their Fig. 8 and Table S3). The second group is 10 samples with ages of 420 Ma or younger (excluding the eclogite), with similar ages (from 420 ± 9 to 412 ± 14 Ma) and yielding similar P–T conditions of 1.36–1.77 GPa (mean of 1.55 ± 0.28 GPa, 2 SD) at 660–920 ∘C (mean of 778 ± 154 ∘C, 2 SD), again without any clear temporal trend (Fig. 8 of Wang et al., 2022, their Table S3). It is true that the ratios for the latter group may be slightly colder than the former group (mean of 555 ± 60 ∘C GPa−1, 2 SD, before 420 Ma and mean of 505 ± 100 ∘C GPa−1, 2 SD, after 420 Ma). By contrast the eclogite records an age of 411 ± 8 Ma, essentially identical to the second group of samples but records P–T conditions of 2.4 ± 0.15 GPa at 830 ± 50 ∘C with of 343 ∘C GPa−1. Thus, the proposed change in thermal gradients could be an artefact of tectonic pressure due to steepening of the subduction zone, with the eclogite recording isothermal compression of > 0.8 GPa at 411 ± 8 Ma. Notably, both explanations rely on the single eclogite sample. Although more data are required to distinguish between the alternative hypotheses, this example illustrates the need to consider all viable explanations when interpreting data rather than concentrating on a single (preferred) model.
As an example of the evolution of ideas in relation to variations in tectonic pressure, consider the spectacular lower crustal rocks of the Bergen Arcs that preserve the conversion of granulite to eclogite. Since the proposal by Austrheim (1987), many have considered this conversion to be related to fluid ingress along shear zones with the degree of overprinting related to the availability of fluid rather than differences in P–T conditions. By contrast, Putnis et al. (2021) recently proposed that fluid ingress through seismic fractures, shear zones and along the foliation created a self-sustaining positive feedback loop between reaction progress and the increase in porosity and permeability in these structural sites, which promoted further ingress of fluid and more reaction, creating a heterogeneous network of weak zones within the strong host. In this model, it is the resulting variations in local pressure that lead to contrasting mineral assemblages between the host and eclogite at the same depth and temperature conditions, and, as reaction progresses, the proportion of eclogite to the host granulite increases and densifies the lower crust. Subsequent modelling has shown this postulate to be plausible (Moulas et al., 2022).
Variations in tectonic pressure potentially have serious implications for eclogite facies metamorphism. For example, is subduction of continental lithosphere to mantle depths a real or apparent phenomenon? At a minimum, it no longer seems useful to follow the lithostatic assumption in the interpretation of orogenic metamorphism – metamorphic pressure and structural depth should be treated as independent variables. Instead, as proposed by Tajčmanová et al. (2021), our community should concentrate on quantifying the magnitude of deviations from lithostatic pressure, since those that are outside of the uncertainty (± 0.2 GPa) will record the tectonic overpressure!
One goal of eclogite studies is to better understand the physical conditions that control processes in subduction zones, including transport of fluids at high pressure; the relationship between metamorphic reactions, fluids and earthquakes; and the generation of melts. During subduction, as P–T conditions change, silicate-buffered fluids vary dramatically in composition and properties, including solute content, density, degree of polymerization and viscosity (Hack et al., 2007; Johnson et al., 2021). At temperatures below the wet solidus minerals become more soluble in aqueous fluid as pressure increases, whereas at temperatures above the wet solidus silicate melts can accommodate more H2O in their structure. Enhanced mutual solubility implies that aqueous fluids and silicate melts become increasingly similar until pressure exceeds the second critical endpoint or critical curve for the silicate–H2O system. Here, miscibility yields a supercritical fluid that varies continuously from an aqueous fluid at lower temperatures and solute content of < 30 % to a supercritical fluid whose properties become closer to those of hydrous melt with increasing solute content at higher temperatures. As a result, subduction zone fluids are categorized into shallow and deep varieties with distinct properties (Manning and Frezzotti, 2020).
6.1 Fluids during subduction
At the present day, fluid-present melting occurs in the mantle wedge above a subducting slab related to hydrate breakdown reactions in downgoing oceanic crustal materials and, especially, the underlying serpentinized mantle, liberating the C-O-H-S fluids involved in the generation of arc magmas (Manning and Frezzotti, 2020; Duan et al., 2022). In particular, the fluid released from serpentinites interacts with the oceanic crustal materials as it migrates along high-permeability pathways generated by deformation localized at lithological boundaries within the slab and at the slab top (Angiboust et al., 2014). However, the mechanism by which porosity networks form to allow for fluid to drain to these high-permeability pathways is poorly understood but is important because the porosity is likely to vary during the transformation and deformation of the slab, as shown for the basaltic crust by Rogowitz and Huet (2021), and episodic drainage events may influence the recurrence of megathrust earthquakes (Yoshida et al., 2023).
Detailed studies, such as that by Plümper et al. (2017) in the Erro–Tobbio metaserpentinites in the Ligurian Alps of Italy, have demonstrated that porosity generation is directly linked to prograde hydrate breakdown reactions that give rise to variations in fluid pressure, forcing the fluid to organize into vein networks from the grain to outcrop scale. Further, thermodynamic modelling has shown that reactive transport and vein widening led to hydraulic fracturing and fluid escape (Huber et al., 2022), with fluxes potentially sufficient to drain the subducting lithosphere. By contrast, other examples of exhumed ocean crust in the Western Alps contain spectacular vugs partially filled with eclogite facies minerals (Angiboust and Raimondo, 2022). These features require closed-system production of a limited volume of fluid during conversion of an HP blueschist mineral assemblage to a denser eclogite facies mineral assemblage under fluid-saturated conditions prior to reaching peak P–T of 2.6–2.9 GPa at 530–570 ∘C. For the vugs to have survived, the host rock must have had a low permeability (< 10−22 m2) and escaped a strong mylonitic overprint. Such local porosity could allow for trapped fluid to be transported deep into Earth's mantle. This contrast in behaviour leaves us with a question – how much fluid is drained from the subducting lithosphere into the mantle wedge, and how much is retained beyond the prograde dehydration window and returned to the mantle? The answer has implications for the production of juvenile crust at arcs and the global volatile budget and, in particular, as to whether the deep water cycle is in secular equilibrium (Hermann and Lakey, 2021).
Fluids and intermediate-depth earthquakes
Earthquakes are limited to < 70 km depth except for regions associated with subduction. However, it remains uncertain what mechanism allows for intermediate-depth (70 to 300 km) earthquakes to occur during subduction. We have long thought that hydrate breakdown reactions in the upper (basaltic) portion of the subducting slab trigger these earthquakes (Kirby et al., 1996; Peacock and Wang, 1999; Hacker et al., 2003b; Abers et al., 2013; Ferrand et al., 2017). Furthermore, the variable distribution and depth of earthquakes may be related to changes in fluid release and fluid pressure associated with prograde metamorphism to eclogite during subduction under different thermal gradients (Qu et al., 2023).
During the last decade a variety of field-based, experimental and modelling studies have confirmed the general relationship between eclogitization and brittle deformation. However, it still remains unclear whether a period of metastability corresponding to a reaction overstep of several hundred megapascals leads to fast transformation and brittle failure (Malvoisin et al., 2020; Yamato et al., 2022), whether earthquake-related brittle failure allows for fluid ingress into dry but potentially reactive crust (Austrheim et al., 2017; Jamtveit et al., 2018, 2019), or whether phenomena such as tectonic tremors and slow slip as well as earthquakes are related to high fluid pressures (Taetz et al., 2018; Marguin and Simpson, 2023; Muñoz-Montecinos et al., 2023). One intriguing observation from some HP eclogites is rhythmic oscillatory zoning of major and trace elements preserved in garnet (Garciá-Casco et al., 2002; Angiboust et al., 2014; Viete et al., 2018). One explanation for such zoning patterns is that they relate to episodic fluid infiltration events generated by the reaction history at depth in the subducting slab (Angiboust et al., 2014). Alternatively, these zoning patterns have been interpreted as recording growth–dissolution cycles driven by pressure changes (Garciá-Casco et al., 2002; Viete et al., 2018) that could have occurred over a period of < 300 000 years (Viete et al., 2018). Such short timescale variations in pressure may be more consistent with fluctuations in pore fluid pressure linked to seismically induced switches from locally undrained to drained conditions (Viete et al., 2018), rather than multiple small-scale exhumation–burial events related to tectonic rupture of the subducting slab (Garciá-Casco et al., 2002), and thus they may provide insight into earthquake recurrence rates. Given the human consequences of earthquakes, achieving a better understanding of their causes and consequences is a major goal in Earth science over the next decade.
6.2 Supercritical fluid and melt around the metamorphic peak and during exhumation
When partial melting occurs during the burial and exhumation cycle is variable according to the specific circumstances. Although prograde melting can occur under the low- gradients that characterize subduction, as evidenced by multiphase solid inclusions (nanogranites) in peak eclogite facies minerals (Ferrero et al., 2015), for rocks that reach HP–UHP conditions, substantial partial melting more commonly occurs during exhumation (Johnson et al., 2021). Furthermore, during high-temperature decompression from UHP to HP metamorphic conditions, a solute-rich supercritical fluid formed by dehydroxylation of nominally anhydrous minerals (NAMs) can evolve to hydrous melt, which has implications for the generation of leucosome in eclogite (Wang et al., 2017, 2020).
Garnet in UHP eclogites commonly contains multiphase solid inclusions (MSIs) that are considered to represent either melt (Gao et al., 2012; Chen et al., 2014) or supercritical fluid (Ferrando et al., 2005; Wang et al., 2016) captured around the metamorphic peak or during exhumation. Typically, these MSIs show clear evidence of post-entrapment decrepitation. An interesting exception to this generalization has been described by Borghini et al. (2023) in eclogites from the Saidenbach Reservoir locality in the Erzgebirge of the Bohemian Massif, where garnets contain MSIs with negative crystal shapes and granitic compositions that have been interpreted as melt trapped around the metamorphic peak. Based on a detailed geochemical study, Borghini et al. (2023) interpret the melt to have been derived from the surrounding microdiamond-bearing metasedimentary rocks. Furthermore, based on trace element compositional similarity with local metasomatized mantle peridotite and pyroxenite, these authors posit that melt with a composition like the Saidenbach MSIs drained from the subducting continental crust and metasomatized the overlying Variscan mantle wedge.
More commonly, based on field studies, experimental petrology and phase equilibrium modelling, dehydroxylation of NAMs and partial melting occur during exhumation, and based on numerical modelling, the presence of melt is considered to be important in facilitating exhumation of subducted continental crust from HP–UHP metamorphic conditions (e.g. Johnson et al., 2021; Labrousse et al., 2011, 2015; Sizova et al., 2012; Wang et al., 2014). If peak pressure conditions were fluid deficient or absent, melting during exhumation can occur due to breakdown of hydrates and/or exsolution of water from NAMs and/or infiltration of water. Hydrate breakdown melting is more frequently cited than fluid-present melting, but petrographic evidence is rarely provided in support. NAMs dissolve water during subduction (tens to hundreds of parts per million, depending on P, T and composition) and exsolve water during exhumation (Wang et al., 2018). Thus, it is likely that dehydroxylation of NAMs during exhumation is important, particularly where there is no petrographic evidence of hydrate breakdown melting at HP–UHP conditions and no isotope record of fluid infiltration (e.g. Wang et al., 2017, 2020). Counterintuitively, in collisional orogens evidence of melting is more commonly found in the eclogites that form a minor component of a subducted continental margin rather than in the volumetrically dominant felsic gneisses. However, this observation is consistent with differences in the intrinsic H2O content of mafic and felsic rocks (Schorn, 2023).
One mechanism by which melting can occur in eclogite is the incongruent melting of omphacite, which may have been overlooked in outcrops lacking detailed microstructural studies. Omphacite typically has water contents from several hundred up to several thousand parts per million (ppm) at UHP conditions (e.g. Katayama et al., 2006; Skogby et al., 2016; Wang et al., 2018, and references therein). Therefore, omphacite is potentially an important source of fluid during exhumation and melting, in addition to any hydrous minerals that may be present. Feng et al. (2021) presented a detailed petrochemical study of grain scale leucosome pockets inferred to have crystallized in situ within UHP eclogite in the central Sulu Belt, China. These authors combined petrological observations with estimated leucosome compositions, constraints on the P–T conditions and timing of leucosome formation to characterize the earliest stage of partial melting in UHP eclogite. For the first time, this study demonstrated that omphacite was the primary contributor to melt formation, with only a minor contribution from phengite, zoisite and quartz (Feng et al., 2021). Although the melt volume is likely to be small, omphacite breakdown melting during exhumation should be common, with important implications for weakening and the mechanisms of exhumation of deeply subducted crust.
In UHP eclogite in the central Sulu Belt, leucosome sheets and composite granite–quartz veins crystallized from 3.5 to 2.2 GPa with decreasing age, well above the critical curve for felsic compositions, consistent with an origin from an evolving solute-rich supercritical fluid during decompression (Wang et al., 2017, 2020). Furthermore, it is plausible that leucosome in other UHP metamorphic belts formed by crystallization from either a solute-rich supercritical fluid or hydrous melt itself derived from an evolving solute-rich supercritical fluid during exhumation. Examples include migmatitic paragneisses at the eastern margin of the Northeast Greenland Eclogite Province (3.6 GPa and 970 ∘C; Lang and Gilotti, 2007, 2015); diamondiferous quartzofeldspathic rocks from the Saxonian Erzgebirge, Germany (∼ 7 GPa, > 1200 ∘C; Massonne, 2003; Massonne and Fockenberg, 2012); and paragneisses from the Kokchetav Massif, Kazakhstan (> 4.5 GPa, 1000 ∘C; Stepanov et al., 2014, 2016).
In the Western Gneiss Region of Norway, migmatitic continental crust including eclogite has been interpreted to record fluid-present melting coeval with peak P–T conditions (> 2.5 GPa, ∼ 800 ∘C; Ganzhorn et al., 2014; Labrousse et al., 2011, 2015). However, the fluid-attending peak metamorphism was more likely to have been solute-rich supercritical fluid that could have increased in volume during exhumation and evolved to a hydrous melt at P < 1.5 GPa. Such an evolution is consistent with zircon U–Pb geochronology and trace element concentrations that show earlier leucosome crystallized in equilibrium with garnet in the absence of plagioclase, whereas later leucosome crystallized in the absence of garnet but in the presence of plagioclase is consistent with an evolution from higher to lower pressure (Gordon et al., 2013). Similarly, a recent study of scapolite pegmatites from the northern segment of the Western Gneiss Region (peak conditions up to 4 GPa and 850 ∘C; Bryden and Jamieson, 2020) considers a possible supercritical fluid source in the petrogenesis of the precursor melts, even though crystallization occurred at much shallower crustal depths under amphibolite facies conditions.
6.3 Fluids and deep earthquakes
Deep earthquakes (300–700 km) occur at depths where brittle failure is thought to be impossible. The prevailing interpretation of this seismicity is that it is generated by the volume change associated with the metastable phase transformation of olivine to spinel (e.g. Green and Burnley, 1989; Gasc et al., 2022; Levitas, 2022), and, in the absence of evidence of fluids at these depths, any possible connection between fluids and deep earthquakes had not been considered. Recently, however, using new subduction zone thermal models, slab earthquake locations, and phase relations of hydrated or carbonated slab mantle and crust, Shirey et al. (2021) explored whether slab fluids can plausibly be transported to depths of > 300 km. Based on the textural characteristics of and the mineral inclusions in diamonds, these authors have shown that fluids were present at the same depths as the earthquake foci. They further argue that decarbonization/melting reactions in the slab crust and hydrate breakdown reactions in the slab mantle provided fluids that cause or are related to the generation of deep earthquakes. This significant step towards a common mechanism for subduction zone earthquakes at all depths should be a focus for further study.
To prosper as a community, our studies of eclogites will need to fit into national science priorities. Increasingly, these priorities relate to the major challenges facing humankind, including exploring and exploiting the resources necessary to support the prosperity of a growing global population while ensuring minimal environmental harm; ensuring safety from natural hazards and, in particular, improving our ability to make accurate predictions of earthquakes, tsunamis and volcanic eruptions; and developing a better understanding of Earth's evolving climate and its drivers while identifying solutions to global warming, particularly in relation to sequestration of carbon dioxide. There is no doubt that the community of researchers working on eclogites can contribute significantly to several of these priorities while also maintaining a substantial research output regarding the more academic aspects of eclogite petrogenesis. Of particular interest currently is fluid cycling between Earth's surface and the deep mantle in a fully coupled system.
The dataset used in Fig. 1, with minor corrections, is from Brown and Johnson (2019c) (available from the author).
The author has declared that there are no competing interests.
Publisher’s note: Copernicus Publications remains neutral with regard to jurisdictional claims in published maps and institutional affiliations.
This article is part of the special issue “(Ultra)high-pressure metamorphism, from crystal to orogenic scale”. It is a result of the 14th International Eclogite Conference (IEC-14) held in Paris and Lyon, France, 10–13 July 2022.
This article is based on an invited talk presented at the 14th International Eclogite Conference; preparation was not supported by grant funds. The author thanks Tim Johnson for comments on the first draft of the manuscript and preparation of the figure. Samuel Angiboust, Antonio García-Casco and Hans-Peter Schertl provided constructive comments during review, leading to an improved article after revision. Nonetheless, the author is responsible for any misrepresentations or infelicities that may persist.
This paper was edited by Samuel Angiboust and reviewed by Hans-Peter Schertl and Antonio García-Casco.
Abers, G. A., Nakajima, J., van Keken, P. E., Kita, S., and Hacker, B. R.: Thermal–petrological controls on the location of earthquakes within subducting plates, Earth Planet. Sc. Lett., 369, 178–187, https://doi.org/10.1016/j.epsl.2013.03.022, 2013.
Agard, P., Yamato, P., Jolivet, L., and Burov, E.: Exhumation of oceanic blueschists and eclogites in subduction zones: Timing and mechanisms, Earth Sc. Rev., 92, 53–79, https://doi.org/10.1016/j.earscirev.2008.11.002, 2009.
Agard, P., Plunder, A., Angiboust, S., Bonnet, G., and Ruha, J.: The subduction plate interface: rock record and mechanical coupling (from long to short timescales), Lithos, 320/321, 537–566, https://doi.org/10.1016/j.lithos.2018.09.029, 2018.
Agard, P., Soret, M., Bonnet, G., Ninkabou, D., Plunder, A., Prigent, C., and Yamato, P.: Subduction and obduction processes: The fate of oceanic lithosphere revealed by blueschists, eclogites, and ophiolites, in: Compressional Tectonics: Plate Convergence to Mountain Building, edited by: Catlos, E. J. and Çemen, I., American Geophysical Union Geophysical Monograph, Vol. 277, John Wiley & Sons, Inc., https://doi.org/10.1002/9781119773856.ch02, 2023.
Alvaro, M., Mazzucchelli, M. L., Angel, R. J., Murri, M., Campomenosi, N., Scambelluri, M., Nestola, F., Korsakov, A., Tomilenko, A. A., Marone, F., and Morana, M.: Fossil subduction recorded by quartz from the coesite stability field, Geology, 48, 24–28, https://doi.org/10.1130/G46617.1, 2020.
Alvaro, M., Angel, R. J., and Nestola, F.: Inclusions in diamonds probe Earth's chemistry through deep time, Commun. Chem., 5, 1–3, https://doi.org/10.1038/s42004-022-00627-1, 2022.
Angel, R. J., Mazzucchelli, M. L., Alvaro, M., Nimis, P., and Nestola, F.: Geobarometry from host-inclusion systems: the role of elastic relaxation, Am. Mineral., 99, 2146–2149, 2014.
Angel, R. J., Mazzucchelli, M. L., Alvaro, M., and Nestola, F.: EosFit-Pinc: A simple GUI for host-inclusion elastic thermobarometry, Am. Mineral., 102, 1957–1960, https://doi.org/10.2138/am-2017-6190, 2017.
Angel, R. J., Murri, M., Mihailova, B., and Alvaro, M.: Stress, strain and Raman shifts, Z. Krist.-Cryst. Mater., 234, 129–140, https://doi.org/10.1515/zkri-2018-2112, 2019.
Angiboust, S. and Raimondo, T.: Permeability of subducted oceanic crust revealed by eclogite-facies vugs, Geology, 50, 964–968, https://doi.org/10.1130/G50066.1, 2022.
Angiboust, S., Pettke, T., de Hoog, J. C. M., Caron, B., and Oncken, O.: Channelized fluid flow and eclogite-facies metasomatism along the subduction shear zone, J. Petrol., 55, 883–916, https://doi.org/10.1093/petrology/egu010, 2014.
Ashley, K. T., Webb, L. E., Spear, F. S., and Thomas, J. B.: P-T-D histories from quartz: a case study of the application of the TitaniQ thermobarometer to progressive fabric development in metapelites, Geochem. Geophy. Geosy., 14, 3821–3843, https://doi. org/10.1002/ggge.20237, 2013.
Aulbach, S. and Smart, K. A.: Petrogenesis and geodynamic significance of xenolithic eclogites, Annu. Rev. Earth Pl. Sc., 51, 521–549, https://doi.org/10.1146/annurev-earth-031621-112904, 2023.
Austrheim, H.: Eclogitization of lower crustal granulites by fluid migration through shear zones, Earth Planet. Sc. Lett., 81, 221–232, https://doi.org/10.1016/0012-821X(87)90158-0, 1987.
Austrheim, H., Dunkel, K. G., Plümper, O., Ildefonse, B., Liu, Y., and Jamtveit, B: Fragmentation of wall rock garnets during deep crustal earthquakes, Sci. Adv., 3, e1602067, https://doi.org/10.1126/sciadv.1602067, 2017.
Auzanneau, E., Schmidt, M. W., Vielzeuf, D., and Connolly, J. A. D.: Titanium in phengite: a geobarometer for high temperature eclogites, Contrib. Mineral. Petrol., 159, 1–24, https://doi.org/10.1007/s00410-009-0412-7, 2010.
Baldwin, S. L., Schönig, J., Gonzalez, J. P., Davies, H., and von Eynatten, H.: Garnet sand reveals rock recycling processes in the youngest exhumed high-and ultrahigh-pressure terrane on Earth, P. Natl. Acad. Sci. USA, 118, e2017231118, https://doi.org/10.1073/pnas.2017231118, 2021.
Baes, M., Sobolev, S., Gerya, T., and Brune, S.: Plume-induced subduction initiation: Single-slab or multi-slab subduction?, Geochem. Geophy. Geosy., 21, e2019GC008663, https://doi.org/10.1029/2019GC008663, 2020.
Baes, M., Sobolev, S., Gerya, T., Stern, R., and Brune, S.: Plate motion and plume-induced subduction initiation, Gondwana Res., 98, 277–288, https://doi.org/10.1016/j.gr.2021.06.007, 2021.
Berg, J.-P. and Gerya, T. V.: The role of viscous heating in Barrovian metamorphism of collisional orogens: thermomechanical models and application to the Lepontine Dome in the Central Alps, J. Metamorph. Geol., 23, 75–95, https://doi.org/10.1111/j.1525-1314.2005.00563.x, 2005.
Berman, R. G.: Internally-consistent thermodynamic data for minerals in the system Na2O-K2O-CaO-MgO-FeO-Fe2O3-Al2O3-SiO2-TiO2-H2O-CO2, J. Petrol., 29, 445–522, https://doi.org/10.1093/petrology/29.2.445, 1988.
Berman, R. G.: Thermobarometry using multi-equilibrium calculations; a new technique, with petrological applications, Can. Mineral., 29, 833–855, 1991.
Borghini, A., Nicoli, G., Ferrero, S., O'Brien, P. J., Laurent, O., Remusat, L., Borghini, G., and Milani, S.: The role of continental subduction in mantle metasomatism and carbon recycling revealed by melt inclusions in UHP eclogites, Sci. Adv., 9, eabp9482, https://doi.org/10.1126/sciadv.abp9482, 2023.
Bovay, T., Lanari, P., Rubatto, D., Smit, M., and Piccoli, F.: Pressure–temperature–time evolution of subducted crust revealed by complex garnet zoning (Theodul Glacier Unit, Switzerland), J. Metamorph. Geol., 40, 175–206, https://doi.org/10.1111/jmg.12623, 2022.
Bonazzi, M., Angel, R. J., Gilio, M., Mazzucchelli, M., and Alvaro, M.: Garnet EoS: a critical review and synthesis, Contrib. Mineral. Petrol., 177, 54, https://doi.org/10.1007/s00410-022-01918-5, 2022.
Bras, E., Yamato, P., Schmalholz, S. M., Duretz, T., and Podladchikov, Y. Y.: Eclogitisation of dry and impermeable granulite by fluid flow with reaction-induced porosity: Insights from hydro-chemical modelling, Earth Planet. Sc. Lett., 617, 118256, https://doi.org/10.1016/j.epsl.2023.118256, 2023.
Brown, M.: Metamorphic conditions in orogenic belts: A record of secular change, Int. Geol. Rev., 49, 193–234, https://doi.org/10.2747/0020-6814.49.3.193, 2007.
Brown, M.: The spatial and temporal patterning of the deep crust and implications for the process of melt extraction, Philos. T. R. Soc. A, 368, 11–51, https://doi.org/10.1098/rsta.2009.0200, 2010.
Brown, M. and Johnson, T.: Secular change in metamorphism and the onset of global plate tectonics, Am. Mineral., 103, 181–196, https://doi.org/10.2138/am-2018-6166, 2018.
Brown, M. and Johnson, T.: Time's arrow, time's cycle: granulite metamorphism and geodynamics, Mineral. Mag., 83, 323–338, https://doi.org/10.1180/mgm.2019.19, 2019a.
Brown, M. and Johnson, T.: Metamorphism and the evolution of subduction on Earth, Am. Mineral., 104, 1065–1082, https://doi.org/10.2138/am-2019-6956, 2019b.
Brown, M. and Johnson, T.: Global age, temperature and pressure data for secular change in metamorphism, EarthChem Library [data set], https://doi.org/10.1594/IEDA/111316, 2019c.
Brown, M., Averkin, Y. A., McLellan, E. L., and Sawyer, E. W.: Melt segregation in migmatites, J. Geophys. Res., 100, 15,655–15,679, https://doi.org/10.1029/95JB00517, 1995.
Brown, M., Kirkland, C., and Johnson, T.: Secular evolution of geodynamics since the Paleoarchean: significant change at the dawn of the Phanerozoic, Geology, 48, 488–492, https://doi.org/10.1130/G47417.1, 2020.
Brown, M., Johnson, T., and Spencer, C.: Secular changes in metamorphism and metamorphic cooling rates track the evolving plate tectonic regime on Earth, J. Geol. Soc. Lond., 179, jgs2022-050, https://doi.org/10.1144/jgs2022-050, 2022.
Brueckner, H. K., Gilotti, J. A., and Nutman, A. P.: Caledonian eclogite-facies metamorphism of Early Proterozoic protoliths from the North-East Greenland Eclogite Province, Contrib. Mineral. Petrol., 130, 103–120, https://doi.org/10.1007/s004100050353, 1998.
Bryden, C. D. and Jamieson, R. A.: Scapolite pegmatite from the Nordøyane ultra-high pressure domain, Western Gneiss Region, Norway: Partial melting driven by infiltration of mantle-derived fluid, Lithos, 364–365, 105546, https://doi.org/10.1016/j.lithos.2020.105546, 2020.
Bulanova, G. P., Walter, M. J., Smith, C. B., Kohn, S. C., Armstrong, L. S., Blundy, J., and Gobbo, L.: Mineral inclusions in sublithospheric diamonds from Collier 4 kimberlite pipe, Juina, Brazil: subducted protoliths, carbonated melts and primary kimberlite magmatism, Contrib. Mineral. Petrol., 160, 489–510, https://doi.org/10.1007/s00410-010-0490-6, 2010.
Campomenosi, N., Angel, R. J., Alvaro, M., and Mihailova, B.: Resetting of zircon inclusions in garnet: Implications for elastic thermobarometry, Geology, 51, 23–27, https://doi.org/10.1130/G50431.1, 2023a.
Campomenosi, N., Angel, R.J., Alvaro, M., and Mihailova, B.: Quartz-in-garnet (QuiG) under pressure: insights from in situ Raman spectroscopy, Contrib. Mineral. Petrol., 178, 44, https://doi.org/10.1007/s00410-023-02026-8, 2023.
Castelli, D., Rolfo, F., Groppo, C., and Compagnoni, R.: Impure marbles from the UHP Brossasco-Isasca Unit (Dora-Maira Massif, western Alps): evidence for Alpine equilibration in the diamond stability field and evaluation of the X(CO2) fluid evolution, J. Metamorph. Geol., 25, 587–603, https://doi.org/10.1111/j.1525-1314.2007.00716.x, 2007.
Chapman, T., Clarke, G. L., and Daczko, N. R.: The role of buoyancy in the fate of ultra-high-pressure eclogite, Sci. Rep., 9, 19925, https://doi.org/10.1038/s41598-019-56475-y, 2019.
Chen, Y. X., Zheng, Y. F., Gao, X. Y., and Hu, Z.: Multiphase solid inclusions in zoisite-bearing eclogite: Evidence for partial melting of ultrahigh-pressure metamorphic rocks during continental collision, Lithos, 200–201, 1–21, https://doi.org/10.1016/j.lithos.2014.04.004, 2014.
Chen, Y. X., Zhou, K., He, Q., Zheng, Y. F., Schertl, H. P., and Chen, K.: First finding of continental deep subduction in the Sesia Zone of Western Alps and implications for subduction dynamics, Nat. Sci. Rev., 10, nwad023, https://doi.org/10.1093/nsr/nwad023, 2023.
Chopin, C.: Coesite and pure pyrope in high-grade blueschists of the Western Alps: a first record and some consequences, Contrib. Mineral. Petrol., 86, 107–118, https://doi.org/10.1007/BF00381838, 1984.
Chowdhury, P., Chakraborty, S., Gerya, T. V., Cawood, P. A., and Capitanio, F. A.: Peel-back controlled lithospheric convergence explains the secular transitions in Archean metamorphism and magmatism, Earth Planet. Sc. Lett., 538, 116224, https://doi.org/10.1016/j.epsl.2020.116224, 2020.
Chowdhury, P., Chakraborty, S., and Gerya, T. V.: Time will tell: Secular change in metamorphic timescales and the tectonic implications, Gondwana Res., 93, 291–310, https://doi.org/10.1016/j.gr.2021.02.003, 2021.
Cionoiu, S., Moulas, E., Stünitz, H., and Tajčmanová, L.: Locally resolved stress-state in samples during experimental deformation: Insights into the effect of stress on mineral reactions, J. Geophys. Res.-Sol. Ea., 127, e2022JB024814, https://doi.org/10.1029/2022JB024814, 2022.
Cisneros, M. and Befus, K. S.: Applications and limitations of elastic thermobarometry: insights from elastic modeling of inclusion-host pairs and example case studies, Geochem. Geophy. Geosy., 21, e2020GC009231, https://doi.org/10.1029/2020GC009231, 2020.
Cisneros, M., Behr, W. M., Platt, J. P., and Anczkiewicz, R.: Quartz-in-garnet barometry constraints on formation pressures of eclogites from the Franciscan Complex, California, Contrib. Mineral. Petrol., 177, 1–23, https://doi.org/10.1007/s00410-021-01876-4, 2022.
Coleman, R. G., Lee, D. E., Beatty, L. B., and Brannock, W. W.: Eclogites and eclogites: Their differences and similarities, Geol. Soc. Am. Bull., 76, 483–508, https://doi.org/10.1130/0016-7606(1965)76[483:EAETDA]2.0.CO;2, 1965.
Condie, K. C., Aster, R. C., and van Hunen, J.: A great thermal divergence in the mantle beginning 2.5 Ga: Geochemical constraints from greenstone basalts and komatiites, Geosci. Front., 7, 543–553, https://doi.org/10.1016/j.gsf.2016.01.006, 2016.
Connolly, J. A. D.: Multivariable phase diagrams; an algorithm based on generalized thermodynamics, Am. J. Sc., 290, 666–718, https://doi.org/10.2475/ajs.290.6.666, 1990.
Connolly, J. A. D.: Computation of phase equilibria by linear programming: a tool for geodynamic modeling and its application to subduction zone decarbonation, Earth Planet. Sc. Lett., 236, 524–541, https://doi.org/10.1016/j.epsl.2005.04.033, 2005.
Connolly, J. A. D. and Podladchikov, Y. Y.: Fluid flow in compressive tectonic settings: Implications for midcrustal seismic reflectors and downward fluid migration, J. Geophys. Res.-Sol. Ea., 109, B04201, https://doi.org/10.1029/2003JB002822, 2004.
de Capitani, C. and Brown, T. H.: The computation of chemical equilibrium in complex systems containing non-ideal solutions, Geochim. Cosmochim. Ac., 51, 2639–2652, https://doi.org/10.1016/0016-7037(87)90145-1, 1987.
de Capitani, C. and Petrakakis, K.: The computation of equilibrium assemblage diagrams with Theriak/Domino software, Am. Mineral., 95, 1006–1016, https://doi.org/10.2138/am.2010.3354, 2010.
Dewey, J. F. and Bird, J. M.: Mountain belts and the new global tectonics, J. Geophys. Res., 75, 2625–2647, https://doi.org/10.1029/JB075i014p02625, 1970.
Dobrzhinetskaya, L. F., O'Bannon III, E. F., and Sumino, H.: Non-cratonic diamonds from UHP metamorphic terranes, ophiolites and volcanic sources, Rev. Mineral. Geochem., 88, 191–255, https://doi.org/10.2138/rmg.2022.88.04, 2022.
Doucet, L. S., Li, Z. X., El Dien, H. G., Pourteau, A., Murphy, J. B., Collins, W. J., Mattielli, N., Olierook, H. K. H., Spencer, C. J., and Mitchell, R. N.: Distinct formation history for deep-mantle domains reflected in geochemical differences, Nat. Geosci., 13, 511–515, https://doi.org/10.1038/s41561-020-0599-9, 2020.
Duan, W.-Y., Li, X.-P., Schertl, H.-P., and Willner, A. P.: C-O-H-S fluids released by oceanic serpentinite in subduction zones: Implications for arc-magma oxidation, Earth Planet. Sc. Lett., 594, 117709, https://doi.org/10.1016/j.epsl.2022.117709, 2022.
Duesterhoeft, E. and Lanari, P.: Iterative thermodynamic modelling – Part 1: A theoretical scoring technique and a computer program (Bingo-Antidote), J. Metamorph. Geol., 38, 527–551, https://doi.org/10.1111/jmg.12538, 2020.
Dunlap, W. J.: Nature's diffusion experiment: the cooling-rate cooling-age correlation, Geology, 28, 139–142, https://doi.org/10.1130/0091-7613(2000)28<139:NDETCC>2.0.CO;2, 2000.
Ehlers, A. M., Zaffiro, G., Angel, R. J., Boffa-Ballaran, T., Carpenter, M. A., Alvaro, M., and Ross, N. L.: Thermoelastic properties of zircon: Implications for geothermobarometry, Am. Mineral., 107, 74–81, https://doi.org/10.2138/am-2021-7731, 2022.
Enami, M., Nishiyama, T., and Mouri, T.: Laser Raman microspectrometry of metamorphic quartz: A simple method for comparison of metamorphic pressures, Am. Mineral., 92, 1303–1315, https://doi.org/10.2138/am.2007.2438, 2007.
Ernst, W. G.: Metamorphic zonations on presumably subducted lithospheric plates from Japan, California and the Alps, Contrib. Mineral. Petrol., 34, 43–59, https://doi.org/10.1007/BF00376030, 1971.
Ernst, W. G.: Blueschist metamorphism and PT regimes in active subduction zones, Tectonophysics, 17, 255–272, https://doi.org/10.1016/0040-1951(73)90006-1, 1973.
Evans, B. W., Trommsdorff, V., and Richter, W.: Petrology of an eclogite-metarodingite suite at Cima di Gagnone, Ticino, Switzerland, Am. Mineral., 64, 15–31, 1979.
Evans, K. A., Frost, B. R., Reddy, S. M., and Brown, T. C.: Causes, effects, and implications of the relationships amongst fluids, serpentinisation, and alloys, Lithos, 446–447, 107132, https://doi.org/10.1016/j.lithos.2023.107132, 2023.
Fan, X., Chu, X., Cao, W., and Zou, Y.: Local rapid exhumation during the long-lived Grenville orogeny, in: Laurentia: Turning Points in the Evolution of a Continent, edited by: Whitmeyer, S. J., Williams, M. L., Kellett, D. A., and Tikoff, B., Geol. Soc. Am. Mem., 220, 307–330, https://doi.org/10.1130/2022.1220(18), 2023.
Faryad, S. W., Baldwin, S. L., Jedlicka, R., and Ježek, J.: Two stage garnet growth in coesite eclogite from the southeastern Papua New Guinea (U)HP terrane, Contrib. Mineral. Petrol., 174, 73, https://doi.org/10.1007/s00410-019-1612-4, 2019.
Feng, P., Wang, L., Brown, M., Johnson, T. E., Kylander-Clark, A., and Piccoli, P. M.: Partial melting of ultrahigh-pressure eclogite by omphacite-breakdown facilitates exhumation of deeply-subducted crust, Earth Planet. Sc. Lett., 554, 116664, https://doi.org/10.1016/j.epsl.2020.116664, 2021.
Ferrand, T. P., Hilairet, N., Incel, S., Deldicque, D., Labrousse, L., Gasc, J., Renner, J., Wang, Y., Green II, H. W., and Schubnel, A.: Dehydration-driven stress transfer triggers intermediate-depth earthquakes, Nat. Commun., 8, 15247, https://doi.org/10.1038/ncomms15247, 2017.
Ferrando, S., Frezzotti, M. L., Dallai, L., and Compagnoni, R.: Multiphase solid inclusions in UHP rocks (Su-Lu, China): Remnants of supercritical silicate-rich aqueous fluids released during continental subduction, Chem. Geol., 223, 68–81, https://doi.org/10.1016/j.chemgeo.2005.01.029, 2005.
Ferrero, S., Wunder, B., Walczak, K., O'Brien, P. J., and Ziemann, M. A.: Preserved near ultrahigh-pressure melt from continental crust subducted to mantle depths, Geology, 43, 447–450, https://doi.org/10.1130/G36534.1, 2015.
Ferry, J. M. and Watson, E. B.: New thermodynamic models and revised calibrations for the Ti-in-zircon and Zr-in-rutile thermometers, Contrib. Mineral. Petrol., 154, 429–437, https://doi.org/10.1007/s00410-007-0201-0, 2007.
Forster, M., Lister, G., Compagnoni, R., Giles, D., Hills, Q., Betts, P., Beltrando, M., and Tamagno, E.: Mapping of oceanic crust with “HP” to “UHP” metamorphism: The Lago di Cignana Unit (Western Alps), https://openresearch-repository.anu.edu.au/handle/1885/36551 (last access: 14 February 2023), 2004.
Ganzhorn, A. C., Labrousse, L., Prouteau, G., Leroy, C., Vrijmoed, J. C., Andersen, T. B., and Arbaret, L.: Structural, petrological and chemical analysis of syn-kinematic migmatites: Insights from the Western Gneiss Region, Norway, J. Metamorph. Geol., 32, 647–673, https://doi.org/10.1111/jmg.12084, 2014.
Gao, X. Y., Zheng, Y. F., and Chen, Y. X.: Dehydration melting of ultrahigh-pressure eclogite in the Dabie orogen: Evidence from multiphase solid inclusions in garnet, J. Metamorph. Geol., 30, 193–212, https://https://doi.org/10.1111/j.1525-1314.2011.00962.x, 2012.
Garciá-Casco, A., Torres-Roldán, R. L., Millán, G., Monié, P., and Schneider, J.: Oscillatory zoning in eclogitic garnet and amphibole, Northern Serpentinite Melange, Cuba: a record of tectonic instability during subduction?, J. Metamorph. Geol., 20, 581–598, https://doi.org/10.1046/j.1525-1314.2002.00390.x, 2002.
Gasc, J., Daigre, C., Moarefvand, A., Deldicque, D., Fauconnier, J., Gardonio, B., Madonna, C., Burnley, P., and Schubnel, A.: Deep-focus earthquakes: From high-temperature experiments to cold slabs, Geology, 50, 1018–1022, https://doi.org/10.1130/G50084.1, 2022.
Gerya, T. V.: Tectonic overpressure and underpressure in lithospheric tectonics and metamorphism, J. Metamorph. Geol., 33, 785–800, https://https://doi.org/10.1111/jmg.12144, 2015.
Gerya, T. V., Stöckhert, B., and Perchuk, A. L.: Exhumation of high-pressure metamorphic rocks in a subduction channel: A numerical simulation, Tectonics, 21, 1056, https://doi.org/10.1029/2002TC001406, 2002.
Gerya, T. V., Stern, R. J., Baes, M., Sobolev, S. V., and Whattam, S. A.: Plate tectonics on the Earth triggered by plume-induced subduction initiation, Nature, 527, 221–225, https://doi.org/10.1038/nature15752, 2015.
Ghignone, S., Scaramuzzo, E., Bruno, M., and Livio, F.: A new UHP unit in the Western Alps: First occurrence of coesite from the Monviso Massif (Italy), Am. Mineral., 108, 1368–1375, https://doi.org/10.2138/am-2022-8621, 2023.
Gilio, M., Angel, R. J., and Alvaro, M.: Elastic geobarometry: How to work with residual inclusion strains and pressures, Am. Mineral., 106, 1530–1533, https://doi.org/10.2138/am-2021-7928, 2021.
Gilio, M., Scambelluri, M., Angel, R. J., and Alvaro, M.: The contribution of elastic geothermobarometry to the debate on HP versus UHP metamorphism, J. Metamorph. Geol., 40, 229–242, https://doi.org/10.1111/jmg.12625, 2022.
Godard, G.: Eclogites and their geodynamic interpretation: a history, J. Geodynam., 32, 165–203, https://doi.org/10.1016/S0264-3707(01)00020-5, 2001.
Gonzalez, J. P., Mazzucchelli, M. L., Angel, R. J., and Alvaro, M.: Elastic geobarometry for anisotropic inclusions in anisotropic host minerals: Quartz-in-zircon, J. Geophys. Res.-Sol. Ea., 126, e2021JB022080, https://doi.org/10.1029/2021JB022080, 2021.
Gordon, S. M., Whitney, D. L., Teyssier, C., and Fossen, H.: U-Pb dates and trace-element geochemistry of zircon from migmatite, Western Gneiss Region, Norway: Significance for history of partial melting in continental subduction, Lithos, 170–171, 35–53, https://doi.org/10.1016/j.lithos.2013.02.003, 2013.
Gilotti, J. A., McClelland, W. C., Schorn, S., Compagnoni, R., and Coble, M. A.: Provenance, protolith and metamorphic ages of jadeite-bearing orthogneiss and host paragneiss at Tavagnasco, the Sesia Zone, Lower Aosta Valley, Italy, Eur. J. Mineral., submitted, 2023.
Giuntoli, F., Lanari, P., Burn, M., Kunz, B. E., and Engi, M.: Deeply subducted continental fragments – Part 2: Insight from petrochronology in the central Sesia Zone (western Italian Alps), Solid Earth, 9, 191–222, https://doi.org/10.5194/se-9-191-2018, 2018.
Green II, H. W. and Burnley, P. C.: A new self-organizing mechanism for deep-focus earthquakes, Nature, 341, 733–737, https://doi.org/10.1038/341733a0, 1989.
Hack, A. C., Thompson, A. B., and Aerts, M.: Phase relations involving hydrous silicate melts, aqueous fluids, and minerals, Rev. Mineral. Geochem., 65, 129–185, https://doi.org/10.2138/rmg.2007.65.5, 2007.
Hacker, B. R. and Gerya, T. V.: Paradigms, new and old, for ultrahigh-pressure tectonism, Tectonophysics, 603, 79–88, https://doi.org/10.1016/j.tecto.2013.05.026, 2013.
Hacker, B. R., Ratschbacher, L., Webb, L., Ireland, T., Walker, D., and Shuwen, D.: U Pb zircon ages constrain the architecture of the ultrahigh-pressure Qinling–Dabie Orogen, China, Earth Planet. Sc. Lett., 161, 215–230, https://doi.org/10.1016/S0012-821X(98)00152-6, 1998.
Hacker, B. R., Abers, G. A., and Peacock, S. M.: Subduction factory: 1. Theoretical mineralogy, densities, seismic wave speeds, and H2O contents, J. Geophys. Res., 108, 2029, https://doi.org/10.1029/2001JB001127, 2003a.
Hacker, B. R., Peacock, S. M., Abers, G. A., and Holloway, S. D.: Subduction factory 2. Are intermediate-depth earthquakes in subducting slabs linked to metamorphic dehydration reactions?, J. Geophys. Res.-Sol. Ea., 108, 2030, https://doi.org/10.1029/2001JB001129, 2003b.
Hanchar, J. M. and Hoskin, P. W. O.: Zircon, Rev. Mineral. Geochem., 53, 500 pp., 2003.
Harte, B. and Richardson, S.: Mineral inclusions in diamonds track the evolution of a Mesozoic subducted slab beneath West Gondwanaland, Gondwana Res., 21, 235–245, https://doi.org/10.1016/j.gr.2011.07.001, 2012.
Hermann, J.: Experimental evidence for diamond-facies metamorphism in the Dora-Maira massif, Lithos, 70, 163–182, https://doi.org/10.1016/S0024-4937(03)00097-5, 2003.
Hermann, J. and Lakey, S.: Water transfer to the deep mantle through hydrous, Al-rich silicates in subduction zones, Geology, 49, 911–915, https://doi.org/10.1130/G48658.1, 2021.
Hermann, J. and Rubatto, D.: Subduction of continental crust to mantle depth: geochemistry of ultrahigh-pressure rocks, Treat. Geochem. 4, 309–340, https://doi.org/10.1016/B978-0-08-095975-7.00309-0, 2014.
Herzberg, C.: Understanding the Paleoproterozoic Circum-Superior Large Igneous Province constrains the thermal properties of Earth's mantle through time, Precambrian Res., 375, 106671, https://doi.org/10.1016/j.precamres.2022.106671, 2022a.
Herzberg, C.: Thermal history of the Earth: Reply to “Less is not always more: A more inclusive data-filtering approach to secular mantle cooling” by Ross N. Mitchell and Jérôme Ganne, Precambrian Res., 381, 106844, https://doi.org/10.1016/j.precamres.2022.106844, 2022b.
Herzberg, C., Condie, K., and Korenaga, J.: Thermal history of the Earth and its petrological expression, Earth Planet. Sc. Lett., 292, 79–88, https://doi.org/10.1016/j.epsl.2010.01.022, 2010.
Holder, R. M., Viete, D. R., Brown, M., and Johnson, T. E.: Metamorphism and the evolution of plate tectonics, Nature, 572, 378–381, https://doi.org/10.1038/s41586-019-1462-2, 2019.
Holland, T. J. B., Green, E. C. R., and Powell, R.: A thermodynamic model for feldspars in KAlSi3O8–NaAlSi3O8–CaAl2Si2O8 for mineral equilibrium calculations, J. Metamorph. Geol., 40, 587–600, https://doi.org/10.1111/jmg.12639, 2022.
Huber, K., Vrijmoed, J. C., and John, T.: Formation of olivine veins by reactive fluid flow in a dehydrating serpentinite, Geochem. Geophy. Geosy., 23, e2021GC010267, https://doi.org/10.1029/2021GC010267, 2022.
Jackson, M. G. and Macdonald, F. A.: Hemispheric geochemical dichotomy of the mantle is a legacy of austral supercontinent assembly and onset of deep continental crust subduction, AGU Advances, 3, e2022AV000664, https://doi.org/10.1029/2022AV000664, 2022.
Jamtveit, B., Ben-Zion, Y., Renard, F., and Austrheim, H.: Earthquake-induced transformation of the lower crust, Nature, 556, 487–491, https://doi.org/10.1038/s41586-018-0045-y, 2018.
Jamtveit, B., Petley-Ragan, A., Incel, S., Dunkel, K. G., Aupart, C., Austrheim, H., Corfu, F., Menegon, L., and Renard, F.: The effects of earthquakes and fluids on the metamorphism of the lower continental crust, J. Geophys. Res.-Sol. Ea., 124, 7725–7755, https://doi.org/10.1029/2018JB016461, 2019.
Jiang, K., Wang, J., Kusky, T., Polat, A., Deng, H., and Wang, L.: Neoarchean seafloor hydrothermal metamorphism of basalts in the Zanhuang ophiolitic mélange, North China Craton, Precambrian Res., 347, 105832, https://doi.org/10.1016/j.precamres.2020.105832, 2020.
Johnson, T. E., Brown, M., Kaus, B., and VanTongeren, J. A.: Delamination and recycling of Archaean crust caused by gravitational instabilities, Nat. Geosci., 7, 47–52, https://doi.org/10.1038/ngeo2019, 2014.
Johnson, T. E., Yakymchuk, C., and Brown, M.: Crustal melting and suprasolidus phase equilibria: From first principles to the state-of-the-art, Earth Sci. Rev., 221, 103778, https://doi.org/10.1016/j.earscirev.2021.103778, 2021.
Jolivet, L., Faccenna, C., Goffé, B., Burov, E., and Agard, P.: Subduction tectonics and exhumation of high-pressure metamorphic rocks in the Mediterranean orogens, Am. J. Sci., 303, 353–409, https://doi.org/10.2475/ajs.303.5.353, 2003.
Katayama, I., Nakashima, S., and Yurimoto, H.: Water content in natural eclogite and implication for water transport into the deep upper mantle, Lithos, 86, 245–259, https://doi.org/10.1016/j.lithos.2005.06.006, 2006.
Kellett, D. A., Weller, O. M., Zagorevski, A., and Regis, D.: A petrochronological approach for the detrital record: Tracking mm-sized eclogite clasts in the northern Canadian Cordillera, Earth Planet. Sc. Lett., 494, 23–31, https://doi.org/10.1016/j.epsl.2018.04.036, 2018.
Kerswell, B. C., Kohn, M. J., and Gerya, T. V.: Computing rates and distributions of rock recovery in subduction zones, Geochem. Geophy. Geosy., 24, e2022GC010834, https://doi.org/10.1029/2022GC010834, 2023.
Kirby, S., Engdahl, E. R., and Denlinger, R.: Intermediate-depth intraslab earthquakes and arc volcanism as physical expressions of crustal and uppermost mantle metamorphism in subducting slabs, Geophys. Monogr. Ser., 96, 195–214, https://doi.org/10.1029/GM096p0195, 1996.
Kohn, M. J.: “Thermoba-Raman-try”: Calibration of spectroscopic barometers and thermometers for mineral inclusions, Earth Planet. Sc. Lett., 388, 187–196, https://doi.org/10.1016/j.epsl.2013.11.054, 2014.
Kohn, M. J.: A refined zirconium-in-rutile thermometer, Am. Mineral., 105, 963–971, https://doi.org/10.2138/am-2020-7091, 2020.
Kohn, M. J., Engi, M., and Lanari, P.: Petrochronology: Methods and applications, Rev. Mineral. Geochem., 83, 575 pp., https://doi.org/10.2138/rmg.2017.83.1, 2017.
Kohn, M. J., Mazzucchelli, M. L., and Alvaro, M.: Elastic Thermobarometry, Annu. Rev. Earth Pl. Sc., 51, 331–66, https://doi.org/10.1146/annurev-earth-031621-112720, 2023.
Korhonen, F. J., Brown, M., Clark, C., and Bhattacharya, S.: Osumilite–melt interactions in ultrahigh temperature granulites: phase equilibria modelling and implications for the P–T–t evolution of the Eastern Ghats Province, India, J. Metamorph. Geol., 31, 881–907, https://doi.org/10.1111/jmg.12049, 2013.
Kurz, W., Handler, R., and Bertoldi, C.: Tracing the exhumation of the Eclogite Zone (Tauern Window, Eastern Alps) by 40Ar 39Ar dating of white mica in eclogites, Swiss J. Geosci., 101, 191–206, https://doi.org/10.1007/s00015-008-1281-1, 2008.
Kylander-Clark, A. R. C., Hacker, B. R., and Mattinson, C. G.: Size and exhumation rate of ultrahigh-pressure terranes linked to orogenic stage, Earth Planet. Sc. Lett., 321, 115–120, https://doi.org/10.1016/j.epsl.2011.12.036, 2012.
Labrousse, L., Prouteau, G., and Ganzhorn, A. C.: Continental exhumation triggered by partial melting at ultrahigh pressure, Geology, 39, 1171–1174, https://doi.org/10.1130/G32316.1, 2011.
Labrousse, L., Duretz, T., and Gerya, T.: H2O-fluid-saturated melting of subducted continental crust facilitates exhumation of ultrahigh-pressure rocks in continental subduction zones, Earth Planet. Sc. Lett., 428, 151–161, https://doi.org/10.1016/j.epsl.2015.06.016, 2015.
Lallemand, S. and Arcay, D.: Subduction initiation from the earliest stages to self-sustained subduction: Insights from the analysis of 70 Cenozoic sites, Earth Sci. Rev., 221, 103779, https://doi.org/10.1016/j.earscirev.2021.103779, 2021.
Lanari, P., and Engi, M.: Local bulk composition effects on metamorphic mineral assemblages, Rev. Mineral. Geochem., 83, 55–102, https://doi.org/10.2138/rmg.2017.83.3, 2017.
Lanari, P. and Hermann, J.: Iterative thermodynamic modelling – Part 2: Tracing equilibrium relationships between minerals in metamorphic rocks, J. Metamorph. Geol., 39, 651–674, https://doi.org/10.1111/jmg.12575, 2021.
Lang, H. M. and Gilotti, J. A.: Partial melting of metapelites at ultrahigh-pressure conditions, Greenland Caledonides, J. Metamorph. Geol., 25, 129–147, https://doi.org/10.1111/j.1525-1314.2006.00687.x, 2007.
Lang, H. M. and Gilotti, J. A.: Modeling the exhumation path of partially melted ultrahigh-pressure metapelites, North-East Greenland Caledonides, Lithos, 226, 131–146, https://doi.org/10.1016/j.lithos.2014.10.010, 2015.
Lappin, M. A. and Smith, D. C.: Mantle-equilibrated orthopyroxene-eclogite pods from the Basal Gneisses in the Selje District, Western Norway, J. Petrol., 19, 530–584, https://doi.org/10.1093/petrology/19.3.530, 1978.
Levitas, V. I.: Resolving puzzles of the phase transformation-based mechanism of the strong deep-focus earthquake, Nat. Commun., 13, 6291, https://doi.org/10.1038/s41467-022-33802-y, 2022.
Li, X., Zhang, L., Wei, C., Bader, T., and Guo, J.: Cold subduction recorded by the 1.9 Ga Salma eclogite in Belomorian Province (Russia), Earth Planet. Sc. Lett., 602, 117930, https://doi.org/10.1016/j.epsl.2022.117930, 2023.
Liu, F. L. and Liou, J. G.: Zircon as the best mineral for P–T–time history of UHP metamorphism: A review on mineral inclusions and U–Pb SHRIMP ages of zircons from the Dabie-Sulu UHP rocks, J. Asian Earth Sci., 40, 1–39, https://doi.org/10.1016/j.jseaes.2010.08.007, 2011.
Liu, L., Zhang, J. F., Cao, Y. T., Green II, H. W., Yang, W. Q., Xu, H. J., Liao, X. Y., and Kang, L.: Evidence of former stishovite in UHP eclogite from the South Altyn Tagh, western China, Earth Planet. Sc. Lett., 484, 353–362, https://doi.org/10.1016/j.epsl.2017.12.023, 2018.
Liu, Y. B., Mitchell, R. N., Brown, M., Johnson, T., and Pisarevsky, S.: Linking metamorphism and plate boundaries over the past two billion years, Geology, 50, 631–635, https://doi.org/10.1130/G49637.1, 2022.
Lu, G., Zhao, L., Chen, L., Wan, B., and Wu, F.-Y.: Reviewing subduction initiation and the origin of plate tectonics: What do we learn from present-day Earth?, Earth Planet Phys., 5, 123–140, https://doi.org/10.26464/epp2021014, 2021.
Luisier, C., Baumgartner, L., Schmalholz, S. M., Siron, G., and Vennemann T.: Metamorphic pressure variation in a coherent Alpine nappe challenges lithostatic pressure paradigm, Nat. Commun., 10, 4734, https://doi.org/10.1038/s41467-019-12727-z, 2019.
Luisier, C., Ballèvre, M., and Duretz, T.: The role of H2O on metamorphism and deformation at high pressure: A combined petrological and thermo-mechanical study based on the Gran Paradiso Unit, Western Alps, Lithos, 446–447, 107123, https://doi.org/10.1016/j.lithos.2023.107123, 2023.
Malvoisin, B., Austrheim, H., Hetényi, G., Reynes, J., Hermann, J., Baumgartner, L. P., and Podladchikov, Y. Y.: Sustainable densification of the deep crust, Geology, 48, 673–677, https://doi.org/10.1130/G47201.1, 2020.
Mancktelow, N. S.: Nonlithostatic pressure during sediment subduction and the development and exhumation of high-pressure metamorphic rocks, J. Geophys. Res.-Sol. Ea., 100, 571–583, https://doi.org/10.1029/94JB02158, 1995.
Mancktelow, N. S.: Tectonic pressure: Theoretical concepts and modelled examples, Lithos, 103, 149–177, https://doi.org/10.1016/j.lithos.2007.09.013, 2008.
Manning, C. E. and Frezzotti, M. L.: Subduction-zone fluids, Elements, 16, 395–400, https://doi.org/10.2138/gselements.16.6.395, 2020.
Manzotti, P., Bosse, V., Pitra, P., Robyr, M., Schiavi, F., and Ballèvre, M.: Exhumation rates in the Gran Paradiso Massif (Western Alps) constrained by in situ U–Th–Pb dating of accessory phases (monazite, allanite and xenotime), Contrib. Mineral. Petrol., 173, 24, https://doi.org/10.1007/s00410-018-1452-7, 2018.
Manzotti, P., Schiavi, F., Nosenzo, F., Pitra, P., and Ballèvre, M.: A journey towards the forbidden zone: a new, cold, UHP unit in the Dora-Maira Massif (Western Alps), Contrib. Mineral. Petrol., 177, 59, https://doi.org/10.1007/s00410-022-01923-8, 2022.
Marguin, V. and Simpson, G.: Influence of fluids on earthquakes based on numerical modeling, J. Geophys. Res.-Sol. Ea., 128, e2022JB025132, https://doi.org/10.1029/2022JB025132, 2023.
Massonne, H.-J.: A comparison of the evolution of diamondiferous quartz-rich rocks from the Saxonian Erzgebirge and the Kokchetav Massif: are so-called diamondiferous gneisses magmatic rocks?, Earth Planet. Sc. Lett., 216, 347–364, https://doi.org/10.1016/S0012-821X(03)00512-0, 2003.
Massonne, H.-J. and Fockenberg, T.: Melting of metasedimentary rocks at ultrahigh pressure – Insights from experiments and thermodynamic calculations, Lithosphere, 4, 269–285, https://doi.org/10.1130/L185.1, 2012.
Mayne, M. J., Moyen, J. F., Stevens, G., and Kaislaniemi, L.: Rcrust: a tool for calculating path-dependent open system processes and application to melt loss, J. Metamorph. Geol., 34, 663–682, https://doi.org/10.1111/jmg.12199, 2016.
Mazzucchelli, M. L., Angel, R. J., and Alvaro, M.: EntraPT: an online platform for elastic geothermobarometry, Am. Mineral., 106, 830–837, https://doi.org/10.2138/am-2021-7693CCBYNCND, 2021.
Melnik, A. E., Skublov, S. G., Rubatto, D., Müller, D., Li, X. H., Li, Q. L., Berezin, A. V., Herwartz, D., and Machevariani, M. M.: Garnet and zircon geochronology of the Paleoproterozoic Kuru-Vaara eclogites, northern Belomorian Province, Fennoscandian Shield, Precambrian Res., 353, 106014, https://doi.org/10.1016/j.precamres.2020.106014, 2021.
Mingardi, G., Campomenosi, N., Gilio, M., Chopin, C., Scambelluri, M., and Alvaro, M.: Elastic thermobarometry of ultrahigh-pressure metapelites from the Brossasco Isasca unit (Dora-Maira Massif, Western Alps), Lithos, 448–449, 107167, https://doi.org/10.1016/j.lithos.2023.107167, 2023.
Moulas, E., Podladchikov, Y. Y., Aranovich, L. Y., and Kostopoulos, D.: The problem of depth in geology: When pressure does not translate into depth, Petrology, 21, 527–538, https://doi.org/10.1134/S0869591113060052, 2013.
Moulas, E., Schmalholz, S., Podladchikov, Y., Tajčmanová, L., Kostopoulos, D., and Baumgartner, L.: Relation between mean stress, thermodynamic, and lithostatic pressure, J. Metamorph. Geol., 37, 1–14, https://doi.org/10.1111/jmg.12446, 2019.
Moulas, E., Kaus, B., and Jamtveit, B.: Dynamic pressure variations in the lower crust caused by localized fluid-induced weakening, Commun. Earth Environ., 3, 157, https://doi.org/10.1038/s43247-022-00478-7, 2022.
Muñoz-Montecinos, J., Angiboust, S., García-Casco, A., and Raimondo, T.: Shattered veins elucidate brittle creep processes in the deep slow slip and tremor region, Tectonics, 42, e2022TC007605, https://doi.org/10.1029/2022TC007605, 2023.
Murri, M., Mazzucchelli, M. L., Campomenosi, N., Korsakov, A. V., Prencipe, M., Mihailova, B. D., Scambelluri, M., Angel, R. J., and Alvaro, M.: Raman elastic geobarometry for anisotropic mineral inclusions, Am. Mineral., 103, 1869–1872, https://doi.org/10.2138/am-2018-6625CCBY, 2018.
Murri, M., Gonzalez, J. P., Mazzucchelli, M. L., Prencipe, M., Mihailova, B., Angel, R. J., and Alvaro, M.: The role of symmetry-breaking strains on quartz inclusions in anisotropic hosts: Implications for Raman elastic geobarometry, Lithos, 422, 106716, https://doi.org/10.1016/j.lithos.2022.106716, 2022.
Ogasawara, Y., Fukasawa, K., and Maruyama, S.: Coesite exsolution from supersilicic titanite in UHP marble from the Kokchetav Massif, northern Kazakhstan, Am. Mineral., 87, 454–461, https://doi.org/10.2138/am-2002-0409, 2002.
O'Neill, C., Lenardic, A., Moresi, L., Torsvik, T. H., and Lee, C. T. A.: Episodic Precambrian subduction, Earth Planet. Sc. Lett., 262, 552–562, https://doi.org/10.1016/j.epsl.2007.04.056, 2007.
O'Neill, C., Marchi, S., Zhang, A., and Bottke, W.: Impact-driven subduction on the Hadean Earth, Nat. Geosci., 10, 793–797, https://doi.org/10.1038/ngeo3029, 2017.
O'Neill, C., Marchi, S., Bottke, W., and Fu, R.: The role of impacts on Archaean tectonics, Geology, 48, 174–178, https://doi.org/10.1130/G46533.1, 2020.
O'Neill, C., Brown, M., Schaefer, B., and Gazi, J. A.: Earth's anomalous middle-age magmatism driven by plate slowdown, Sci. Rep., 12, 10460, https://doi.org/10.1038/s41598-022-13885-9, 2022.
Osborne, Z. R., Thomas, J. B., Nachlas, W. O., Angel, R. J., Hoff, C. M., and Watson, E. B.: TitaniQ revisited: expanded and improved Ti-in-quartz solubility model for thermobarometry, Contrib. Mineral. Petrol., 177, 31, https://doi.org/10.1007/s00410-022-01896-8, 2022.
Palin, R. and White, R.: Emergence of blueschists on Earth linked to secular changes in oceanic crust composition, Nat. Geosci., 9, 60–64, https://doi.org/10.1038/ngeo2605, 2016.
Palin, R. M., Weller, O. M., Waters, D. J., and Dyck, B.: Quantifying geological uncertainty in metamorphic phase equilibria modelling; a Monte Carlo assessment and implications for tectonic interpretations, Geosci. Front., 7, 591–607, https://doi.org/10.1016/j.gsf.2015.08.005, 2016.
Peacock, S. M. and Wang, K.: Seismic consequences of warm versus cool subduction metamorphism: examples from southwest and northeast Japan, Science, 286, 937–939, https://doi.org/10.1126/science.286.5441.937, 1999.
Penniston-Dorland, S. C., Kohn, M. J., and Manning, C. E.: The global range of subduction zone thermal structures from exhumed blueschists and eclogites: Rocks are hotter than models, Earth Planet. Sc. Lett., 428, 243–254, https://doi.org/10.1016/j.epsl.2015.07.031, 2015.
Perchuk A. L., Zakharov V. S., Gerya T. V., and Brown, M.: Hotter mantle but colder subduction in the Precambrian: what are the implications?, Precambrian Res., 330, 20–34, https://doi.org/10.1016/j.precamres.2019.04.023, 2019.
Perraki, M. and Faryad, S. W.: First finding of microdiamond, coesite and other UHP phases in felsic granulites in the Moldanubian Zone: Implications for deep subduction and a revised geodynamic model for Variscan Orogeny in the Bohemian Massif, Lithos, 203, 157–166, https://doi.org/10.1016/j.lithos.2014.05.025, 2014.
Petrini, K. and Podladchikov, Y.: Lithospheric pressure-depth relationship in compressive regions of thickened crust, J. Metamorph. Geol., 18, 67–77, https://doi.org/10.1046/j.1525-1314.2000.00240.x, 2000.
Piccoli, F., Rubatto, D., Ovtcharova, M., Hermann, J., Guillong, M., and Brovarone, A. V.: Dating fluid infiltration and deformation in the subducted ultramafic oceanic lithosphere by perovskite geochronology, Chem. Geol., 615, 121205, https://doi.org/10.1016/j.chemgeo.2022.121205, 2023.
Pleuger, J. and Podladchikov, Y. Y.: A purely structural restoration of the NFP20-East cross section and potential tectonic overpressure in the Adula nappe (central Alps), Tectonics, 33, 656–685, https://doi.org/10.1002/2013TC003409, 2014.
Plümper, O., John, T., Podladchikov, Y. Y., Vrijmoed, J. C., and Scambelluri, M.: Fluid escape from subduction zones controlled by channel-forming reactive porosity, Nat. Geosci., 10, 150–156, https://doi.org/10.1038/ngeo2865, 2017.
Powell, R. and Holland, T. J. B.: An internally consistent dataset with uncertainties and correlations: 3. Applications to geobarometry, worked examples and a computer program, J. Metamorph. Geol., 6, 173–204, https://doi.org/10.1111/j.1525-1314.1988.tb004 15.x, 1988.
Powell, R. and Holland, T. J. B.: Optimal geothermometry and geobarometry, Am. Mineral., 79, 120–133, 1994.
Powell, R. and Holland, T. J. B.: On thermobarometry, J. Metamorph. Geol., 26, 155–179, https://doi.org/10.1111/j.1525-1314.2007.00756.x, 2008.
Powell, R., Holland, T. J. B., and Worley, B.: Calculating phase diagrams involving solid solutions via non-linear equations, with examples using THERMOCALC, J. Metamorph. Geol.,16, 577–588, https://doi.org/10.1111/j.1525-1314.1998.00157.x, 1998.
Powell, R., Evans, K. A., Green, E. C. R., and White, R. W.: On equilibrium in non-hydrostatic metamorphic systems, J. Metamorph. Geol., 36, 419–438, https://doi.org/10.1111/jmg.12298, 2018.
Putnis, A., Moore, J., Prent, A. M., Beinlich, A., and Austrheim, H.: Preservation of granulite in a partially eclogitized terrane: Metastable phenomena or local pressure variations?, Lithos, 400/401, 106413, https://doi.org/10.1016/j.lithos.2021.106413, 2021.
Qu R., Zhu, W., Ji, Y., Xie, C., Zeng, D., and Zhang, F.: Subduction thermal regime, petrological metamorphism and seismicity under the Mariana arc, Sci. Rep., 13, 1948, https://doi.org/10.1038/s41598-023-29004-1, 2023.
Regis, D., Rubatto, D., Darling, J., Cenki-Tok, B., Zucali, M., and Engi, M.: Multiple metamorphic stages within an eclogite-facies terrane (Sesia Zone, Western Alps) revealed by Th–U–Pb petrochronology, J. Petrol., 55, 1429–1456, https://doi.org/10.1093/petrology/egu029, 2014.
Rogowitz, A. and Huet, B.: Evolution of fluid pathways during eclogitization and their impact on formation and deformation of eclogite: A microstructural and petrological investigation at the type locality (Koralpe, Eastern Alps, Austria), Tectonophysics, 819, 229079, https://doi.org/10.1016/j.tecto.2021.229079, 2021.
Rosenfeld, J. L. and Chase, A. B.: Pressure and temperature of crystallization from elastic effects around solid inclusions in minerals?, Am. J. Sci., 259, 519–541, https://doi.org/10.2475/ajs.259.7.519, 1961.
Rubatto, D., Gebauer, D., and Fanning, M.: Jurassic formation and Eocene subduction of the Zermatt–Saas-Fee ophiolites: implications for the geodynamic evolution of the Central and Western Alps, Contrib. Mineral. Petrol., 132, 269–287, https://doi.org/10.1007/s004100050421, 1998.
Rubatto, D., Gebauer, D., and Compagnoni, R.: Dating of eclogite-facies zircons: the age of Alpine metamorphism in the Sesia–Lanzo Zone (Western Alps), Earth Planet. Sc. Lett., 167, 141–158, https://doi.org/10.1016/S0012-821X(99)00031-X, 1999.
Rutland, R. W. R.: Tectonic overpressures, in: Controls of Metamorphism, edited by: Pitcher, W. S. and Flinn, G. W., Oliver & Boyd, Edinburgh, 119–139, 1965.
Schertl, H.-P., Schreyer, W., and Chopin, C.: The pyrope-coesite rocks and their country rocks at Parigi, Dora-Maira Massif, Western Alps: detailed petrography, mineral chemistry and P–T path, Contrib. Mineral. Petrol., 108, 1–21, https://doi.org/10.1007/BF00307322, 1991.
Schönig, J., Meinhold, G., von Eynatten, H., and Lünsdorf, N. K.: Tracing ultrahigh-pressure metamorphism at the catchment scale, Sci. Rep., 8, 2931, https://doi.org/10.1038/s41598-018-21262-8, 2018a.
Schönig, J., Meinhold, G., von Eynatten, H., and Lünsdorf, N. K.: Provenance information recorded bymineral inclusions in detrital garnet, Sed. Geol., 376, 32–49, https://doi.org/10.1016/j.sedgeo.2018.07.009, 2018b.
Schönig, J., von Eynatten, H., Meinhold, G., and Lünsdorf, N. K.: Diamond and coesite inclusions in detrital garnet of the Saxonian Erzgebirge, Germany, Geology, 47, 715–718, https://doi.org/10.1130/G46253.1, 2019.
Schönig, J., von Eynatten, H., Meinhold, G., and Lünsdorf, N. K.: Life-cycle analysis of coesite-bearing garnet, Geol. Mag., 158, 1421–1440, https://doi.org/10.1017/S0016756821000017, 2021.
Schönig, J., von Eynatten, H., Meinhold, G., and Lünsdorf, N. K.: The sedimentary record of ultrahigh-pressure metamorphism: a perspective review, Earth Sci. Rev., 227, 103985, https://doi.org/10.1016/j.earscirev.2022.103985, 2022.
Schorn, S.: Self-induced incipient “eclogitization” of metagranitoids at closed-system conditions, J. Metamorph. Geol., 40, 1271–1290, https://doi.org/10.1111/jmg.12665, 2022.
Schwarzenbach, E. M., Zhong, X., Caddick, M. J., Schmalholz, S. M., Menneken, M., Hecht, L., and John, T.: On exhumation velocities of high-pressure units based on insights from chemical zoning in garnet (Tianshan, NW China), Earth Planet. Sc. Lett., 570, 117065, https://doi.org/10.1016/j.epsl.2021.117065, 2021.
Scibiorski, E., Tohver, E., and Jourdan, F.: Rapid cooling and exhumation in the western part of the Mesoproterozoic Albany-Fraser Orogen, Western Australia, Precambrian Res., 265, 232–248, https://doi.org/10.1016/j.precamres.2015.02.005, 2015.
Shirey, S. B. and Richardson, S. H.: Start of the Wilson cycle at 3 Ga shown by diamonds from subcontinental mantle, Science, 333, 434–36, https://doi.org/10.1126/science.1206275, 2011.
Shirey, S. B., Wagner, L. S., Walter, M. J., Pearson, D. G., and van Keken, P. E.: Slab transport of fluids to deep focus earthquake depths – thermal modeling constraints and evidence from diamonds, AGU Advances, 2, e2020AV000304, https://doi.org/10.1029/2020AV000304, 2021.
Simon, M., Pitra, P., Yamato, P., and Poujol, M.: Isothermal compression of an eclogite from the Western Gneiss Region (Norway), J. Metamorph. Geol., 41, 181–203, https://doi-org/10.1111/jmg.12692, 2023.
Simpson, A., Gilbert, S., Tamblyn, R., Hand, M., Spandler, C., Gillespie, J., Nixon, A., and Glorie, S.: In-situ Lu–Hf geochronology of garnet, apatite and xenotime by LA ICP MS/MS, Chem. Geol., 577, 120299, https://doi.org/10.1016/j.chemgeo.2021.120299, 2021.
Simpson, A., Glorie, Hand, M., Spandler, C., and Gilbert, S.: Garnet Lu-Hf speed dating: A novel method to rapidly resolve polymetamorphic histories, Gondwana Res., 121, 215–234, https://doi.org/10.1016/j.gr.2023.04.011, 2023.
Sizova, E., Gerya, T., Brown, M., and Perchuk, L.: Subduction styles in the Precambrian: Insight from numerical experiments, Lithos, 116, 209–229, https://doi.org/10.1016/j.lithos.2009.05.028, 2010.
Sizova, E., Gerya, T., and Brown, M.: Exhumation mechanisms of melt-bearing ultrahigh pressure crustal rocks during collision of spontaneously moving plates, J. Metamorph. Geol., 30, 927–955, https://doi.org/10.1111/j.1525-1314.2012.01004.x, 2012.
Sizova E., Gerya T., and Brown M.: Contrasting styles of Phanerozoic and Precambrian continental collision, Gondwana Res., 25, 522–545, https://doi.org/10.1016/j.gr.2012.12.011, 2014.
Sizova, E., Hauzenberger, C., Fritz, H., Faryad, S. W., and Gerya, T.: Late orogenic heating of (ultra)high pressure rocks: Slab Rollback vs. Slab Breakoff, Geosciences, 9, 1–28, https://doi.org/10.3390/geosciences9120499, 2019.
Skogby, H., Janák, M., and Broska, I.: Water incorporation in omphacite: concentrations and compositional relations in ultrahigh-pressure eclogites from Pohorje, Eastern Alps, Europ. J. Mineral., 28, 631–639, https://doi.org/10.1127/ejm/2016/0028-2533, 2016.
Smit, K. V., Timmerman, S., Aulbach, S., Shirey, S. B., Richardson, S. H., Phillips, D., and Pearson, D. G.: Geochronology of Diamonds, Rev. Mineral. Geochem., 88, 567–636, https://doi.org/10.2138/rmg.2022.88.11, 2022.
Smith, D. C.: Coesite in clinopyroxene in the Caledonides and its implications for geodynamics, Nature, 310, 641–644, https://doi.org/10.1038/310641a0, 1984.
Smith, E. M., Shirey, S. B., Richardson, S. H., Nestola, F., Bullock, E. S., Wang, J., and Wang, W.: Blue boron-bearing diamonds from Earth's lower mantle, Nature, 560, 84–87, https://doi.org/10.1038/s41586-018-0334-5, 2018.
Smye, A. J. and England, P. C.: Metamorphism and deformation on subduction interfaces: 2. Petrological and tectonic implications, Geochem. Geophy. Geosy., 24, e2022GC010645, https://doi.org/10.1029/2022GC010645, 2023.
Smye, A. J., Marsh, J. H., Vermeesch, P., Garber, J. M., and Stockli, D. F.: Applications and limitations of U-Pb thermochronology to middle and lower crustal thermal histories, Chem. Geol., 494, 1–18, https://doi.org/10.1016/j.chemgeo.2018.07.003, 2018.
Sobolev, N. V. and Shatsky, V. S.: Diamond inclusions in garnet from metamorphic rocks: a new environment for diamond formation, Nature, 343, 742–746, https://doi.org/10.1038/343742a0, 1990.
Sobolev, S. V. and Brown, M.: Surface erosion events controlled the evolution of plate tectonics on Earth, Nature, 570, 52–57, https://doi.org/10.1038/s41586-019-1258-4, 2019.
Spear, F. S., Wolfe, O. M., and Cheney, J. T.: On the interpretation of TitaniQ and ZiR thermobarometry in subduction complexes, Contrib. Mineral. Petrol., 178, 8, https://doi.org/10.1007/s00410-022-01989-4, 2023.
Spencer, C. J., Mitchell, R. N., and Brown, M.: Enigmatic Mid-Proterozoic orogens: Hot, thin, and low, Geophys. Res. Lett., 48, e2021GL093312, https://doi.org/10.1029/2021GL093312, 2021.
Spengler, D. and Alifirova, T. A.: New UHP eclogite in between UHP areas, WGR, Norway, IEC-14, Lyon, France, Unpubl Abstract, 2022.
Spengler, D., Alifirova, T. A., and van Roermund, H. L. M.: Subcratonic and tectonic evolution of pyroxenite and eclogite with lamellar inclusions in garnet, Western Gneiss Region, Norway, J. Petrol., 62, egab008, https://doi.org/10.1093/petrology/egab008, 2021.
Spránitz, T., Szabó, C., Gilio, M., Alvaro, M., Blazeková, M., Konecný, P., Váczi, T., and Berkesi, M.: Estimation of P-T entrapment conditions of a subduction fluid using elastic thermobarometry: A case study from Cabo Ortegal Complex, Spain, Lithos, 448–449, 107171, https://doi.org/10.1016/j.lithos.2023.107171, 2023.
Stepanov, A. S., Hermann, J., Korsakov, A. V., and Rubatto, D.: Geochemistry of ultrahigh-pressure anatexis: fractionation of elements in the Kokchetav gneisses during melting at diamond-facies conditions, Contrib. Mineral. Petrol., 167, 1002, https://doi.org/10.1007/s00410-014-1002-x, 2014.
Stepanov, A. S., Hermann, J., Rubatto, D., Korsakov, A. V., and Danyushevsky, L. V.: Melting history of an ultrahigh-pressure paragneiss revealed by multiphase solid inclusions in garnet, Kokchetav massif, Kazakhstan, J. Petrol., 57, 1531–1554, https://doi.org/10.1093/petrology/egw049, 2016.
Stern, R. J. and Gerya, T.: Subduction initiation in nature and models: A review, Tectonophys, 746, 173–198, https://doi.org/10.1016/j.tecto.2017.10.014, 2018.
Taetz, S., John, T., Bröcker, M., Spandler, C., and Stracke, A.: Fast intraslab fluid-flow events linked to pulses of high pore fluid pressure at the subducted plate, Earth Planet. Sc. Lett., 482, 33–43, https://doi.org/10.1016/j.epsl.2017.10.044, 2018.
Tajčmanová, L., Podladchikov, Y., Powell, R., Moulas, E., Vrijmoed, J. C., and Connolly, J. A. D.: Grain-scale pressure variations and chemical equilibrium in high-grade metamorphic rocks, J. Metamorph. Geol., 32, 195–207, https://doi.org/10.1111/jmg.12066, 2014.
Tajčmanová, L., Vrijmoed, J., and Moulas, E.: Grain-scale pressure variations in metamorphic rocks: implications for the interpretation of petrographic observations, Lithos, 216, 338–351, https://doi.org/10.1016/j.lithos.2015.01.006, 2015.
Tajčmanová, L., Manzotti, P., and Alvaro, M.: Under pressure: high-pressure metamorphism in the Alps, Elements, 17, 17–22, https://doi.org/10.2138/gselements.17.1.17, 2021.
Tamblyn, R., Hasterok, D., Hand, M., and Gard, M.: Mantle heating at ca. 2 Ga by continental insulation: Evidence from granites and eclogites, Geology, 50, 91–95, https://doi.org/10.1130/G49288.1, 2022a.
Tamblyn, R., Hand, M., Simpson, A., Gilbert, S., Wade, B., and Glorie, S.: In situ laser ablation Lu–Hf geochronology of garnet across the Western Gneiss Region: campaign-style dating of metamorphism, J. Geol. Soc Lond., 179, https://doi.org/10.1144/jgs2021-094, 2022b.
Thomas, J. B. and Spear, F. S.: Experimental study of quartz inclusions in garnet at pressures up to 3.0 GPa: Evaluating validity of the quartz-in-garnet inclusion elastic thermobarometer, Contrib. Mineral. Petrol., 173, 1–14, https://doi.org/10.1007/s00410-018-1469-y, 2018.
Thomas, J. B., Watson, E. B., Spear, F. S., Shemella, P. T., Nayak, S. K., and Lanzirotti, A.: TitaniQ under pressure: the effect of pressure and temperature on the solubility of Ti in quartz, Contrib. Mineral. Petrol., 160, 743–759, https://doi-org/10.1007/s00410-010-0505-3, 2010.
Tomkins, H. S., Powell, R., and Ellis, D. J.: The pressure dependence of the zirconium-in-rutile thermometer, J. Metamorph. Geol., 25, 703–713, https://doi.org/10.1111/j.1525-1314.2007.00724.x, 2007.
Tual, L., Smit, M. A., Cutts, J., Kooijman, E., Kielman-Schmitt, M., Majka, J., and Foulds, I.: Rapid, paced metamorphism of blueschists (Syros, Greece) from laser-based zoned Lu-Hf garnet chronology and LA-ICPMS trace element mapping, Chem. Geol., 607, 121003, https://doi.org/10.1016/j.chemgeo.2022.121003, 2022.
van Hunen, J. and van den Berg, A.: Plate tectonics on the early Earth: limitations imposed by strength and buoyancy of subducted lithosphere, Lithos, 103, 217–235, https://doi.org/10.1016/j.lithos.2007.09.016, 2008.
van Keken, P. E., Hacker, B. R., Syracuse, E. M., and Abers, G. A.: Subduction factory: 4. Depth-dependent flux of H2O from subducting slabs worldwide, J. Geophys. Res., 116, B01401, https://doi.org/10.1029/2010jb007922, 2011.
van Keken, P. E., Wada, I., Abers, G. A., Hacker, B. R., and Wang, K.: Mafic high-pressure rocks are preferentially exhumed from warm subduction settings, Geochem. Geophy. Geosy., 19, 2934–2961, https://doi.org/10.1029/2018GC007624, 2018.
van Roermund, H. L. M. and Drury, M. R.: Ultra-high pressure (P > 6 GPa) garnet peridotites in Western Norway: exhumation of mantle rocks from > 185 km depth, Terra Nova, 10, 295–301, https://doi.org/10.1046/j.1365-3121.1998.00213.x, 1998.
Vho, A., Lanari, P., Rubatto, D., and Hermann, J.: Tracing fluid transfers in subduction zones: an integrated thermodynamic and δ18O fractionation modelling approach, Solid Earth, 11, 307–328, https://doi.org/10.5194/se-11-307-2020, 2020.
Vho, A., Rubatto, D., Lanari, P., and Regis, D.: The evolution of the Sesia Zone (Western Alps) from Carboniferous to Cretaceous: insights from zircon and allanite geochronology, Swiss J. Geosci., 113, 24, https://doi.org/10.1186/s00015-020-00372-4, 2020.
Viete, D. R. and Lister, G. S.: On the significance of short-duration regional metamorphism, J. Geol. Soc. Lond., 174, 377–392, https://doi.org/10.1144/jgs2016-060, 2017.
Viete, D. R., Hacker, B. R., Allen, M. B., Seward, G. G. E., Tobin, M. J., Kelley, C. S., Cinque, G., and Duckworth, A. R.: Metamorphic records of multiple seismic cycles during subduction, Sci. Adv., 4, eaaq0234, https://doi.org/10.1126/sciadv.aaq0234, 2018.
Wan, B., Yang, X., Tian, X., Yuan, H., Kirscher, U., and Mitchell, R. N.: Seismological evidence for the earliest global subduction network at 2 Ga ago, Sci. Adv., 6, eabc5491, https://doi.org/10.1126/sciadv.abc5491, 2020.
Wang, H., Xiao, W. J., Windley, B. F., Zhang, Q. W. L., Tan, Z., Wu, C. M., and Shi, M. G.: Diverse P–T–t paths reveal high-grade metamorphosed forearc complexes in NW China, China, J. Geophys. Res.-Sol. Ea., 127, e2022JB024309, https://doi.org/10.1029/2022JB024309, 2022.
Wang, J., Li, X., Ning, W., Kusky, T., Wang, L., Polat, A., and Deng, H.: Geology of a Neoarchean suture: Evidence from the Zunhua ophiolitic mélange of the Eastern Hebei Province, North China Craton, Geol. Soc. Am. Bull., 131, 1943–1964,https://doi.org/10.1130/B35138.1, 2019.
Wang, J.-M., Lanari, P., Wu, F.-Y., Zhang, J.-J., Khanal, G. P., and Yang, L.: First evidence of eclogites overprinted by ultrahigh temperature metamorphism in Everest East, Himalaya: Implications for collisional tectonics on early Earth, Earth Planet. Sc. Lett., 558, 116760, https://doi.org/10.1016/j.epsl.2021.116760, 2021.
Wang, L., Kusky, T. M., Polat, A., Wang, S. J., Jiang, X. F., Zong, K. Q., Wang, J. P., Deng, H., and Fu, J. M.: Partial melting of deeply subducted eclogite from the Sulu orogen in China, Nat. Commun., 5, 5604, https://doi.org/10.1038/ncomms6604, 2014.
Wang, L., Wang, S.-J., Brown, M., Zhang, J. F., Feng, P., and Jin, Z. M.: On the survival of intergranular coesite in UHP eclogite, J. Metamorph. Geol., 36, 173–194, https://doi.org/10.1111/jmg.12288, 2018.
Wang, S., Wang, L., Brown, M., and Feng, P.: Multi-stage barite crystallization in partially melted UHP eclogite from the Sulu belt, China, Am. Mineral., 101, 564–579, https://doi.org/10.2138/am-2016-5384, 2016.
Wang, S.-J., Wang, L., Brown, M., Piccoli, P. M., Johnson, T., Feng, P., Deng, H., Kitajima, K., and Huang, Y.: Composite granite–quartz veins in the Sulu belt, China: evidence of supercritical fluid flow during exhumation of deeply subducted UHP continental crust, China, J. Metamorph. Geol., 35, 601–629, https://doi.org/10.1111/jmg.12248, 2017.
Wang, S.-J., Wang, L., Brown, M., Johnson, T., Piccoli, P., Feng, P., and Wang, Z.-L.: Petrogenesis of leucosome sheets in migmatitic UHP eclogites – Evolution from silicate-rich supercritical fluid to hydrous melt, Lithos, 360/361, 105442, https://doi.org/10.1016/j.lithos.2020.105442, 2020.
Wang, S.-J., Brown, M., Wang, L., Johnson, T. E., Olierook, H. K. H., Kirkland, C. L., Kylander-Clark, A., Evans, N. J., and McDonald, B. J.: Two-stage exhumation of deeply-subducted continental crust: Insight from zircon, titanite and apatite petrochronology, Geol. Soc. Am. Bull., 135, 48–66, https://doi.org/10.1130/B36309.1, 2023.
Wang, Y., Wang, K., He, J., and Zhang, L.: On unusual conditions for the exhumation of subducted oceanic crustal rocks: How to make rocks hotter than models, Earth Planet. Sc. Lett., 615, 118213, https://doi.org/10.1016/j.epsl.2023.118213, 2023.
Weller, O. M., Mottram, C. M., St-Onge, M. R., Möller, C., Strachan, R., Rivers, T., and Copley, A.: The metamorphic and magmatic record of collisional orogens, Nat. Rev. Earth Environ., 2, 781–799, https://doi.org/10.1038/s43017-021-00218-z, 2021.
Wheeler, J.: A unifying basis for the interplay of stress and chemical processes in the Earth: support from diverse experiments, Contrib. Mineral. Petrol., 175, 116, https://doi.org/10.1007/s00410-020-01750-9, 2020.
Willigers, B. J. A., van Gool Willigers, J. A. M., Wijbrans, J. R., Krogstad, E. J., and Mezger, K.: Posttectonic cooling of the Nagssugtoqidian Orogen and a comparison of contrasting cooling histories in Precambrian and Phanerozoic orogens, J. Geol., 110, 503–517, https://doi.org/10.1086/341595, 2002.
Xiang, H. and Connolly, J. A. D.: GeoPS: An interactive visual computing tool for thermodynamic modelling of phase equilibria, J. Metamorph. Geol., 40, 243–255, https://doi.org/10.1111/jmg.12626, 2022.
Xu, Z., Wang, Q., Tang, Z., and Chen, F.: Fabric kinematics of the ultrahigh-pressure metamorphic rocks from the main borehole of the Chinese Continental Scientific Drilling Project: Implications for continental subduction and exhumation, Tectonophysics, 475, 235–250, https://doi.org/10.1016/j.tecto.2009.02.041, 2009.
Yamato, P., Agard, P., Burov, E., Le Pourhiet, L., Jolivet, L., and Tiberi, C.: Burial and exhumation in a subduction wedge: Mutual constraints from thermomechanical modeling and natural P-T-t data (Schistes Lustrés, western Alps), J. Geophys. Res., 112, B07410, https://doi.org/10.1029/2006JB004441, 2007.
Yamato, P., Duretz, T., Baïsset, M., and Luisier, C.: Reaction-induced volume change triggers brittle failure at eclogite facies conditions, Earth Planet. Sc. Lett., 584, 117520, https://doi.org/10.1016/j.epsl.2022.117520, 2022.
Yardley, B. W. and Valley, J. W.: The petrologic case for a dry lower crust, J. Geophys. Res.-Sol. Ea., 102, 12173–12185, https://doi.org/10.1029/97JB00508, 1997.
Yoshida, K., Oyanagi, R., Kimura, M., Plümper, O., Fukuyama, M., and Okamoto, A.: Geological records of transient fluid drainage into the shallow mantle wedge, Sci. Adv., 9, eade6674, https://doi.org/10.1126/sciadv.ade6674, 2023.
Young, D. J. and Kylander-Clark, A. R. C.: Does continental crust transform during eclogite facies metamorphism?, J. Metamorph. Geol., 33, 337–351, https://doi.org/10.1111/jmg.12123, 2015.
Zhao, L., Tyler, I. M., Gorczyk, W., Murdie, R. E., Gessner, K., Lu, Y., Smithies, H., Li, T., Yang, J., Zhan, A., Wan, B., Sun, B., Yuan, H., and the CWAS Group: Seismic evidence of two cryptic sutures in Northwestern Australia: Implications for the style of subduction during the Paleoproterozoic assembly of Columbia, Earth Planet. Sc. Lett., 579, 117342, https://doi.org/10.1016/j.epsl.2021.117342, 2022.
Zhong, X., Vrijmoed, J., Moulas, E., and Tajčmanová, L.: A coupled model for intragranular deformation and chemical diffusion, Earth Planet. Sc. Lett., 474, 387–396, https://doi.org/10.1016/j.epsl.2017.07.005, 2017.
Zou, Y., Mitchell, R. N., Chu, X., Brown, M., Li, Q. L., Zhao, L., and Zhai, M. G.: Surface evolution during the mid-Proterozoic stalled by mantle warming under Columbia–Rodinia, Earth Planet. Sc. Lett., 607, 118055, https://doi.org/10.1016/j.epsl.2023.118055, 2023.
- Abstract
- Introduction
- Thermobarometry, petrochronology and detrital materials
- Modelling subduction zone processes
- Secular change, plate tectonics and diamonds
- Variations in tectonic pressure
- Fluids and melts
- Concluding remarks
- Data availability
- Competing interests
- Disclaimer
- Special issue statement
- Acknowledgements
- Review statement
- References
- Abstract
- Introduction
- Thermobarometry, petrochronology and detrital materials
- Modelling subduction zone processes
- Secular change, plate tectonics and diamonds
- Variations in tectonic pressure
- Fluids and melts
- Concluding remarks
- Data availability
- Competing interests
- Disclaimer
- Special issue statement
- Acknowledgements
- Review statement
- References