the Creative Commons Attribution 4.0 License.
the Creative Commons Attribution 4.0 License.
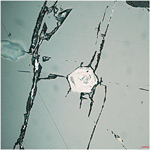
Skogbyite, Zr(Mg2Mn3+4)SiO12, a new zirconium mineral in the braunite group from Långban, Bergslagen, Sweden
Erik Jonsson
Ulf Hålenius
Jaroslaw Majka
Ferdinando Bosi
Skogbyite, ideally Zr(Mg2Mn)SiO12, is a new mineral species (IMA 2023-085) within the braunite group, discovered in a complex metamorphic assemblage from the Långban Fe–Mn oxide deposit, Värmland County, Bergslagen ore province, Sweden. It is named after the Swedish mineralogist Henrik Skogby (b. 1956). The new mineral is hosted by a hausmannite–jacobsite-bearing calcite–dolomite–phlogopite rock that additionally contains pinakiolite, macedonite and sparse baddeleyite. In this assemblage, skogbyite occurs as very small, mostly ≤ 60 µm, rounded to subhedral grey crystals. It is suggested that skogbyite formed during regional metamorphism of a pre-existing, weakly Mn(–Fe)-mineralised carbonate–silicate rock. Optically, the mineral is opaque and bluish grey with a moderate reflectance in reflected polarised light and exhibits weak bireflectance and anisotropy. The Mohs hardness is estimated to be 6–6.5, and it has a calculated density of 4.821 g cm−3. Skogbyite is tetragonal, space group I4, with unit-cell parameters a= 9.4914(4) Å, c= 18.9875(10) Å, V= 1710.52(17) Å3 and Z= 8. Its crystal structure was refined to R1= 0.0460 for 648 unique reflections with I > 2σI, collected utilising MoKα X-ray radiation. The five strongest (calculated) powder X-ray diffraction lines (d in Å, (I), (hkl)) are 2.740, (100), (224); 1.678, (31), (048); 1.431, (16), (264); 1.678, (13), (440); and 5.480, (12), (112).
Electron probe microanalyses combined with single-crystal structure refinement resulted in the following empirical formula: (ZrCeMg0.06Ca0.01Zn0.01Pb0.01)Σ0.87(MnMg1.40FeAl0.09)Σ6.00Si1.04O12 based on 12 O atoms. All Mn in the new mineral is trivalent. Skogbyite is related to gatedalite, Zr(Mn2MnSiO12, by the substitution Mn2+Mg−1. Skogbyite is abbreviated as “Skb”.
- Article
(1628 KB) - Full-text XML
-
Supplement
(223 KB) - BibTeX
- EndNote
Skogbyite, ideally Zr(Mg2MnSiO12, is a new braunite-group mineral from the Långban Fe–Mn deposit (59.86° N, 14.27° E), Filipstad Municipality, Värmland County, in the westernmost part of the ancient and classic Bergslagen ore province in south-central Sweden. The Långban deposit is uniquely mineralogically diverse (e.g. Moore, 1970; Nysten et al., 1999, and references therein; Holtstam et al., 2024), featuring nearly 400 different known species today, of which close to 80 have Långban as their type locality. The deposit primarily comprises dolomite-hosted regionally metamorphosed, deformed and faulted stratabound Fe and Mn oxide ore lenses, with associated skarn bodies, alteration rocks, and variably mineralised veins and fissures. Mining at Långban started with the extraction of Fe ore at least as early as the late 17th century, together with the extraction of Mn ores during the mid-19th to mid-20th centuries and then purely dolomite extraction during the final 15 years until the closure of the mine in 1972 (e.g. Langhof and Österberg, 1999). The deposit is hosted by Palaeoproterozoic (Svecofennian) metamorphosed dolomitic carbonate rocks (marbles) and felsic metavolcanic rocks and is generally considered to have a syngenetic, submarine volcanic–hydrothermal origin (e.g. Holtstam and Mansfeld, 2001; Jonsson, 2004; Jonsson and Billström, 2009, and references therein). Together with related Fe–Mn oxide deposits, all located in the Bergslagen province, it is characterised by significant contents of, for example, As, Ba, Be, Pb and Sb in both ores and associated skarns and other alteration rocks. These all experienced a geologically extended evolution that led to a multitude of minerals that formed during physicochemically variable conditions. Thus, the evolution of the deposit can be coarsely subdivided into four separate main stages (Jonsson, 2004). The initial formation of “primary” minerals, including early carbonates and ore mineral progenitors in a ca. 1.90–1.88 Ga active, mainly felsic volcanic and sub-volcanic shallow marine back-arc setting was followed by polyphase ca. 1.87–1.80 Ga regional (Svecokarelian) upper greenschist-facies-grade to amphibolite-facies-grade metamorphism of this part of Bergslagen (e.g. Allen et al., 1996; Stephens et al., 2009, and references therein). Later, predominantly granitic magmas with minor associated mafic components, intruded at around 1.8 Ga, and, subsequent to that, mainly brittle tectonic processes dominated the following evolution of the deposit. The final fourth stage included the formation of a wide range of minerals in fractures from low-temperature aqueous fluids that redistributed pre-existing elements within the deposit and led to the formation of a plethora of late-stage species (e.g. Nysten et al., 1999; Jonsson and Broman, 2002; Jonsson, 2004; Holtstam et al., 2024).
The new mineral skogbyite is yet another example of the mineralogical diversity and particular geochemical micro-environments that are the hallmarks of this remarkable deposit. After mine closure and the subsequent flooding of the underground workings, only the mine dumps have been accessible for collecting. The sample carrying the new mineral had, as far as is known, already been collected from the Långban mine dumps in the 1950s to 1960s. The new mineral was first noted during studies of oxyborate-bearing assemblages from Långban. It is named after Henrik Skogby (b. 1956),of the Department of Geosciences, Swedish Museum of Natural History (Naturhistoriska riksmuseet), in Stockholm, Sweden, for his significant contributions to mineralogy. These include the characterisation of several new mineral species, two of which (hjalmarite and ferri-taramite) are from Långban-type deposits.
The mineral and its name have been approved by the International Mineralogical Association (IMA) and specifically its Commission on New Minerals, Nomenclature and Classification (CNMNC), in the form of IMA proposal 2023-085 (Jonsson et al., 2024). Holotype material (rock sample, polished thick and thin sections and a mounted single crystal used for microprobe analyses and single-crystal structure refinement) is deposited in the mineral collection of the Swedish Museum of Natural History (Naturhistoriska riksmuseet), Stockholm, Sweden, under catalogue number GEO-NRM20230033.
The new mineral and its overall assemblage were studied by means of optical microscopy (transmitted and reflected polarised light), scanning electron microscopy with energy-dispersive X-ray spectroscopy (SEM-EDS) and back-scattered electron (BSE) imaging, as well as X-ray powder diffraction analysis. Skogbyite occurs as sparse, disseminated, small (mainly ≤ 60 µm, in a singular case up to ca. 120 µm), rounded to angular, anhedral to mainly subhedral grains/crystals (Fig. 1) together with locally coarse-grained pinakiolite [(Mg,Mn2+)2Mn3+(BO3)O2], unevenly distributed irregular and anhedral grains, aggregates and later fracture fillings of Mn–Fe oxides (mainly hausmannite and jacobsite), and minute disseminated euhedral to subhedral macedonite (PbTiO3) crystals in a groundmass dominated by Mn-bearing phlogopite, calcite and dolomite. The skogbyite crystals occur both in isolation in the phlogopite and carbonate-dominated groundmass and as inclusions in the poikiloblastic pinakiolite (Fig. 1). Skogbyite sparsely occurs intimately, or as intergrowths, with the Mn–Fe oxide ore minerals (hausmannite, jacobsite) and is rarely observed directly associated with baddeleyite and/or macedonite. Later-formed species in the assemblage besides Fe–Mn oxides in fractures include sparse, very thin fracture fillings along the basal cleavages of phlogopite in the carbonate–silicate groundmass that consist of anhedral native lead (± litharge). Similarly, very thin fracture fillings also occur in the carbonate groundmass, dominated by hedyphane (Ca2Pb3(AsO4)3Cl).
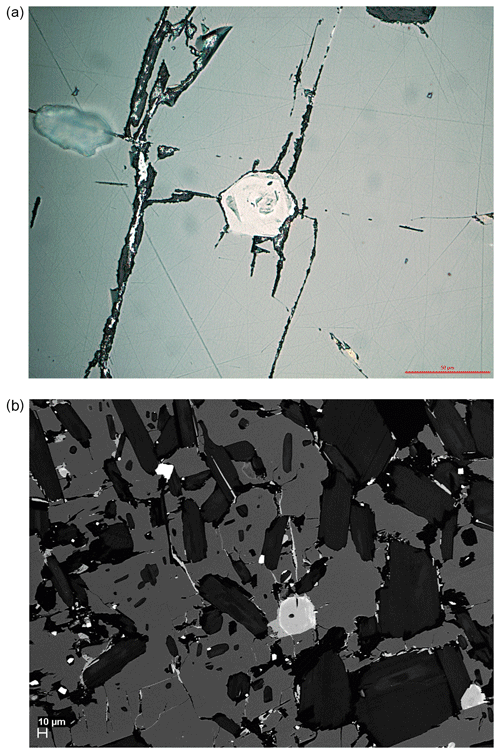
Figure 1(a) Image in plane-polarised reflected light of a subhedral skogbyite crystal with a hexagonal-like outline (centre) included in poikiloblastic pinakiolite. Vague zoning can be seen in the skogbyite. Fracture fillings in the host pinakiolite partly contain Fe–Mn oxides. The scale bar equals 50 µm. (b) High-contrast back-scattered electron (BSE) image showing subhedral skogbyite crystals (medium to light grey with lighter, mainly outer, zones showing Pb enrichment in the centre and lower right) included in poikiloblastic pinakiolite (dark-grey groundmass). The BSE light to BSE white fracture fillings and irregular larger grains are Fe–Mn oxides, while BSE white crystals with a square outline are macedonite. Elongated black inclusions in the pinakiolite are subhedral crystals or aggregates of phlogopite. The scale bar equals 10 µm.
The hardness of skogbyite could not be measured directly due to the very small grain sizes combined with the lack of homogeneous domains for such measurements. By analogy with braunite, its Mohs hardness is estimated as ca. 6–6.5, which is also broadly corroborated by observations of (relative) polishing hardness in reflected, plane-polarised light. The lustre is sub-metallic, and its streak was indicated to be brownish grey in polishing scratches. While the tenacity of skogbyite is brittle, neither cleavage nor parting could be observed. The density could not be measured directly due to the small size and heterogeneity of the crystals and grains. The calculated density is 4.821 g cm−3, on the basis of the empirical formula and unit-cell volume refined from single-crystal X-ray diffraction data. No certain crystal forms could be identified, and no twinning has been observed. The c : a ratio calculated from the single-crystal unit-cell parameters is 0.4999. No fluorescence in ultraviolet light could be observed.
Skogbyite is opaque, and in reflected, plane-polarised light the mineral is bluish grey with moderate reflectance. Due to the minute size of the crystals and their finely and heterogeneously zoned nature, meaningful optical measurements including reflectance values could not be obtained. However, the reflectivity of skogbyite should be similar to that of gatedalite, i.e. between ca. 17 % and 21 % in air (Hålenius and Bosi, 2015), and is slightly lower than the reflectivities of neighbouring Fe–Mn oxide minerals, as observed in plane-polarised light. Skogbyite exhibits weak anisotropy and bireflectance. No pleochroism was observed in the single-crystal section studied. No internal reflections could be observed, not even when utilising very strong light and the oil immersion technique. The crystals are typically variably zoned, visible in back-scattered electron imagery and, to some extent, optically in reflected, plane-polarised light (Fig. 1).
Chemical analyses (19 point analyses in total) were carried out using an electron probe microanalyser (EPMA), a JEOL JXA-8530F Hyperprobe in WDS mode, at 15 kV and 20 nA and with a 1 µm beam diameter. Analytical results and utilised EPMA standards are given in Table 1. No additional REEs were detected. Matrix corrections were performed according to the Armstrong CITZAF method (Armstrong, 1995). Atoms per formula unit (apfu) were based on 12 O atoms; all Mn is considered to be Mn3+ to obtain the smallest Si contents (1.04 apfu). The excellent agreement between the number of electrons per formula unit (epfu) derived from EMPA and SREF (180.74 and 181.64 epfu, respectively) supports the above considerations. There is also a variable presence of Pb-enriched zones in the analysed crystal (see also Fig. 1b). In accordance with the general formula of the braunite-group minerals, [8]A[6]B6SiO12 (Hålenius and Bosi, 2015), the empirical formula of skogbyite is A(ZrCeMg0.06Ca0.01Zn0.01Pb(MnMg1.40FeAl0.09)Σ6.00Si1.04O12.
Table 1Chemical data (in wt %) and analytical standards for EPMA analyses of skogbyite. Averages and ranges from 19 point analyses of a skogbyite crystal.
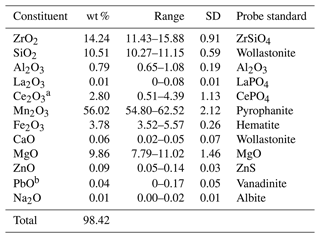
a Ce assumed to be Ce3+ by analogy with all known Ce-bearing minerals in this system. b Only present in certain zones of the mineral.
The simplified formula is thus (Zr,Ce,Mg)(Mn3+,Mg,Fe,Al)6SiO12, while the ideal formula is Zr(Mg2MnSiO12, which requires (in wt %) 21.26 ZrO2, 13.91 MgO, 54.46 Mn2O3 and 10.37 SiO2, total 100.00.
An unpolarised infrared spectrum (Fig. 2) in the range of 650–4000 cm−1 was recorded on a 10 µm × 10 µm area of a skogbyite crystal with a Bruker VERTEX 70 spectrometer attached to a Bruker HYPERION 2000 IR microscope using ATR (attenuated total reflectance) techniques. The spectrum shows a strong band due to Si–O stretching vibrations at 904 cm−1. In braunite (as in the example from India in Fig. 2), this band occurs at higher energies, ∼945 cm−1 (e.g. Chukanov, 2014). This may be ascribed to differences in cation occupancies of the nearest-neighbour sites of the SiO4 tetrahedra. For instance, in skogbyite, the M(1) site is occupied by Zr4+, while in braunite, it is occupied by Mn2+.
6.1 X-ray powder diffraction
X-ray powder diffraction data were not collected due to the very small size of the sufficiently chemically homogeneous skogbyite domains in crystals from the holotype specimen. The powder data were therefore generated in the range of 2θ= 0–90° for the CuKα wavelength using the crystallographic data from the present structural model (see below) and VESTA 3 software (Momma and Izumi, 2011), and they are listed in Table 2. A comparison of the simulated powder diffraction pattern extracted from the observed single-crystal X-ray diffraction intensities with the pattern calculated from the final crystal structure model shows no significant differences. This is a sort of certification that the structural model does not feature any overinterpretation or misconceptions.
6.2 Crystallography and crystal structure refinement
Single-crystal X-ray studies were carried out with a Bruker KAPPA APEX II single-crystal diffractometer equipped with a CCD area detector (6.2 × 6.2 cm2 active detection area, 512 × 512 pixels) and graphite-monochromatised MoKα radiation. A total of 2272 frames were collected using ω and φ scan modes (step = 0.2°, time step = 20 s). Final unit-cell parameters were refined using the Bruker AXS SAINT program from reflections with I > 10σI in the range 7° < 2θ < 69°: a= 9.4914(4) Å, c= 18.9875(10) Å, V= 1710.52(17) Å3, Z= 8 and space group I4.
The intensity data were processed and corrected for Lorentz, polarisation and background effects using the APEX3 software program of Bruker AXS and for absorption using the multiscan method (SADABS). Structure refinement was done using the SHELXL2013 program (Sheldrick, 2015). Starting coordinates were taken from Hålenius and Bosi (2015). Variable parameters were the scale factor; the extinction coefficient; atom coordinates; site-scattering values for M(1), M(2), M(3) and M(4) sites; and atomic-displacement factors. Attempts to refine the extinction coefficient yielded values within its standard uncertainty; thus, it was not refined. Neutral atom-scattering factors were used. In detail, the M(1) site was modelled using the Zr scattering factor. The occupancies of the M(2), M(3) and M(4) sites were obtained considering the presence of Mn versus Mg. The Si position and anion sites were modelled with Si and O scattering factors, respectively, and with a fixed occupancy of 1 because refinement with unconstrained occupancies showed no significant deviations from this value. The crystal structure was refined to R1 = 0.0460 for 658 unique reflections with I > 2σI. Correlations (up to ±0.79) between M(2), M(3) and M(4) site occupancies occurred in the refinement.
Table 3 lists crystal data, data-collection information and refinement details; Table 4 gives the fractional atom coordinates, equivalent isotropic displacement parameters and site occupancies; Table 5 shows selected bond lengths; and Table 6 reports weighted bond valences and bond-valence sums (BVSs) compared to weighted atom valence (mean formal charge, MFC) calculated from the empirical crystal chemical formula. Note that the weighted bond-valence sums appear to be constantly smaller than the MFC values. This general negative deviation of ions from the valence sum rule may be ascribed to the cation–cation electrostatic repulsion across shared edges of the structure (steric strain in the bond valence model), which, along with the Jahn–Teller effect of Mn3+, is also the cause of the polyhedral distortions (Moore and Araki, 1976).
Table 4Fractional atomic coordinates, equivalent isotropic displacement parameters (Å2) and site occupancies (s.o.) for skogbyite.
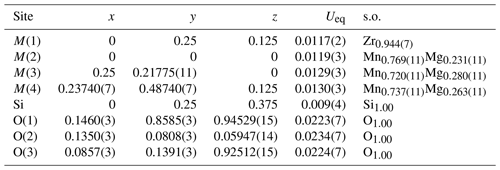
Table 6Weighted bond-valence calculations (valence units) for skogbyite.
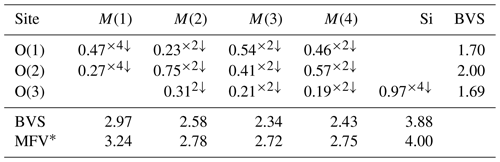
* MFV denotes the mean formal valence from refined site populations. Note that the bond-valence parameters are from Gagné and Hawthorne (2015).
Coordination polyhedra are denoted by the central cation site, for example, the M(2) octahedron. Skogbyite has a braunite-type structure, which is based on the packing of distorted M(1) hexahedra (cubes); distorted M(2), M(3) and M(4) octahedra; and nearly regular SiO4 tetrahedra (Fig. 3). A detailed description of the braunite-type structure is given by Moore and Araki (1976).
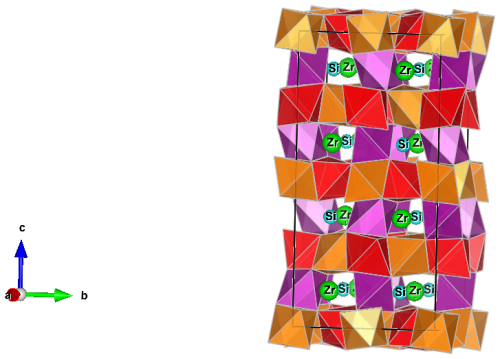
Figure 3The crystal structure of skogbyite projected onto (100). It is characterised by a network of M(2), M(3) and M(4) O6 octahedra occupied by Mn3+ and Mg, forming a three-dimensional framework. Zirconium and Si are in [8]- and [4]-fold coordination, respectively. Orange denotes M(2), red M(3) and purple M(4). The figure was drawn by VESTA (Momma and Izumi, 2011).
Skogbyite, ideally Zr(Mg2MnSiO12, is a member of the braunite mineral group that comprises the species braunite, ideally Mn2+MnSiO12; abswurmbachite, ideally Cu2+MnSiO12; gatedalite, ideally Zr(Mn2MnSiO12; and neltnerite, ideally Ca2+MnSiO12, which all crystallise in the space group I4 (e.g. Hålenius and Bosi, 2015, and references therein). Skogbyite is related to gatedalite by the homovalent substitution Mn2+Mg−1. Table 7 compares skogbyite with gatedalite. The Strunz–Nickel classification for skogbyite is 04.C: metal : oxygen = 2:3.
8.1 Site populations and crystal chemistry
As noted previously, the general formula of the braunite-group minerals may be written as AB6SiO12, where the letter A represents a group of cations (e.g. Mn2+, Ca2+, Mg2+, Cu2+, Zr4+, Ce3+ and Zn2+) accommodated at the eight-coordinated M(1) site, while B represents a group of cations (e.g. Mn2+, Mg, Fe3+, Al, Cu2+ and Ti4+) distributed over three distinct six-coordinated M(2), M(3) and M(4) sites and Si4+ is accommodated at the tetrahedrally coordinated site. The oxygen anions are located at the four-fold-coordinated O(1), O(2) and O(3) sites.
In order to translate the chemical and structural refinement results into site populations at the [6]M(2), [6]M(3) and [6]M(4) sites, we used the program of Wright et al. (2000), which minimises the differences between the formula derived from chemical analysis and that obtained from single-crystal structure refinement. For these optimisations, both bond valences and site scatterings were taken into account. With regard to the [8]M(1) site population, it was fixed with Zr4+, Ce3+, Zn and Pb, in accordance with their relatively large atomic numbers and sizes; the excess of Mg from the sum [M(2) +M(3) +M(4)] = 6 goes to M(1).
The resulting empirical crystal chemical formula is M(1)(ZrCeMg0.06Ca0.01Zn0.01Pb0.01)Σ0.87
M(2)(MnMg0.35Al0.09)Σ2.00
M(3)(MnMg0.55Fe
M(4)(MnMg0.50)Σ2.00Si1.04O12.
Measured and calculated site-scattering values (expressed in terms of the number of electrons per site) are in very good agreement (measured vs. calculated values):
-
M(1) = 37.8(3) vs. 35.1
-
M(2) = 22.0(2) vs. 22.2
-
M(3) = 21.4(2) vs. 21.5
-
M(4) = 21.6(2) vs. 21.7.
The M(1) site is dominated by Zr4+ (A cation), whereas the chemical composition over the octahedrally coordinated sites can be merged as B = [M(2) +M(3) +M(4)] due to the Mg–Mn3+ disorder and to obtain a charge-balanced end-member formula: Zr(Mg2MnSiO12.
8.2 Paragenesis and genesis of skogbyite
Broadly similar to gatedalite (Hålenius and Bosi, 2015), skogbyite occurs in a variably Mn–Fe oxide and carbonate-bearing phyllosilicate-rich alteration rock from Långban, Sweden. As noted above, after initial formation of primary minerals and associated hydrothermal seafloor–sub-seafloor alteration as well as compaction and diagenetic processes, ca. 1.87–1.80 Ga regional (Svecokarelian) metamorphism with associated deformation affected the deposit and its host rocks (e.g. Allen et al., 1996; Stephens et al., 2009, and references therein). In the Långban area, peak regional metamorphic conditions are estimated to have reached ca. 3–4 kbar and 550–600 °C (e.g. Christy and Gatedal, 2005), i.e. uppermost greenschist-facies to lower amphibolite-facies grade. During this stage, the majority of skarn (sensu lato) minerals and early veins were formed and a majority of other alteration rocks and ores were extensively recrystallised and mineralogically modified. Crystal growth by recrystallisation of pre-existing minerals and blastic growth of new ones, stable in the metamorphic pressure–temperature conditions, were common in many of the rocks and mineral assemblage types that make up the Långban deposit and its host rocks. Most typically, such porphyroblasts are abundantly poikilitic (i.e. they are poikiloblasts), as is common in metamorphic rocks in general. It is suggested that skogbyite formed as mainly isolated (micro-)blasts, characteristically subhedral to euhedral, during an early prograde stage of this regional metamorphism of the deposit. Under continued and increasing prograde conditions, possibly aided by fluid-mediated processes mobilising boron, the oxyborate mineral pinakiolite formed as coarse porphyroblasts (poikiloblasts), overgrowing different pre-existing minerals such as abundant micas (phlogopite), Fe and Mn oxides, macedonite, and indeed also skogbyite (Fig. 1). With regard to genetic relations to gatedalite, it is suggested that the more Mg-enriched local environment in the presently studied assemblage, as evidenced by the relative abundance of groundmass dolomite, led to skogbyite formation instead of its Mn2+ analogue gatedalite. The obvious presence of accessible Zr to form new metamorphic minerals in both assemblages is notable and had previously only been evidenced in Långban by the previously mentioned baddeleyite and the calzirtite-group mineral hiärneite, (Ca,Mn2+,Na)2(Zr,Mn3+)5(Sb5+,Ti,Fe3+)2O16 (Holtstam, 1997).
Post-dating the stages of prograde to peak metamorphic mineral growth, the tectonic processes after ca. 1.8 Ga were dominated by brittle deformation, leading to fracturing of pre-existing rocks and mineral assemblages on various scales and to various extents (Jonsson, 2004). In the present assemblage, this is seen in the form of fracturing of the larger pinakiolite poikiloblasts and local infilling of these fractures with, for example, Fe and/or Mn oxide minerals (Fig. 1). Additionally, the occurrence of native lead and arsenate minerals in fracture fillings in the groundmass and along opened basal cleavages of phlogopite reflects late-stage, low-temperature mobilisation of As, Pb and other components, and these minerals are very characteristic of the late-stage fracture-hosted assemblages in the Långban deposit (e.g. Nysten et al., 1999; Jonsson and Broman, 2002; Jonsson, 2004).
A CIF file is deposited in the Supplement section referred to below.
The supplement related to this article is available online at https://doi.org/10.5194/ejm-37-269-2025-supplement.
EJ performed the general mineralogical–paragenetic studies and analyses. UH obtained and interpreted spectroscopic data and prepared material for single-crystal study. JM and EJ performed the chemical analyses. FB collected single-crystal X-ray data and executed the structural refinement. EJ and FB wrote the manuscript in direct collaboration with all authors.
At least one of the (co-)authors is a guest member of the editorial board of European Journal of Mineralogy for the special issue “New minerals: EJM support”. The peer-review process was guided by an independent editor, and the authors also have no other competing interests to declare.
Publisher’s note: Copernicus Publications remains neutral with regard to jurisdictional claims made in the text, published maps, institutional affiliations, or any other geographical representation in this paper. While Copernicus Publications makes every effort to include appropriate place names, the final responsibility lies with the authors.
This article is part of the special issue “New minerals: EJM support”. It is not associated with a conference.
Karin Högdahl (Uppsala University) is heartily thanked for help with mineral separation. We thank the editors for the efficient and careful handling of the manuscript as well as Uwe Kolitsch (Vienna) and the anonymous reviewer for their constructive and helpful comments. The members of IMA-CNMNC are thanked for their useful comments on the original proposal to the commission.
This research was supported by grants from the Italian Ministero dell'Istruzione, dell’Università e della Ricerca (PRIN 2020 “HYDROX – HYDRous- vs. OXo-components in minerals: adding new pieces to the Earth’s H2O cycle puzzle”, project no. 2020WYL4NY).
This paper was edited by Sergey Krivovichev and reviewed by Uwe Kolitsch and one anonymous referee.
Allen, R. L, Lundström, I., Ripa, M., and Christofferson, H.: Facies analysis of a 1.9 Ga, continental margin, back-arc, felsic caldera province with diverse Zn-Pb-Ag-(Cu-Au) sulfide and Fe oxide deposits, Bergslagen region, Sweden, Econ. Geol., 91, 979–1008, https://https://doi.org/10.2113/gsecongeo.91.6.979, 1996.
Armstrong, J. T.: CITZAF: a package of correction programs for the quantitative electron microbeam X-ray analysis of thick polished materials, thin films, and particles, Microbeam. Anal., 4, 177–200, 1995.
Christy, A. and Gatedal, K.: Extremely Pb-rich rock-forming silicates including a beryllian scapolite and associated minerals in a skarn from Långban, Värmland, Sweden, Mineral. Mag., 69, 995–1018, doi.org./10.1180/0026461056960304, 2005.
Chukanov, N. V.: Infrared spectra of mineral species. Extended library. Vol. 1, Springer Dordrecht Heidelberg New York London, 1–1726, https://doi.org/10.1007/978-94-007-7128-4, 2014.
Gagné, O. C. and Hawthorne, F. C.: Comprehensive derivation of bond- valence parameters for ion pairs involving oxygen, Acta Crystallogr. B, 71, 562–578, https://doi.org/10.1107/S2052520615016297, 2015.
Hålenius, U. and Bosi, F.: Gatedalite, Zr(MnMnSiO12, a new mineral species of the braunite group from Långban, Sweden, Mineral. Mag., 79, 625–634, https://doi.org/10.1180/minmag.2015.079.3.08, 2015.
Holtstam, D.: Hiärneite, a new, Zr-Sb oxide mineral isostructural with calzirtite, from Långban, Sweden, Eur. J. Mineral., 9, 843–848, https://doi.org/10.1127/ejm/9/4/0843, 1997.
Holtstam, D. and Mansfeld, J.: Origin of a carbonate-hosted Fe-Mn-(Ba-As-Pb-Sb-W) deposit of Långban-type in central Sweden, Mineral. Dep., 36, 641–657, https://doi.org/10.1007/s001260100183, 2001.
Holtstam, D., Langhof, J., Friis, H., Karlsson, A., and Erambert, M.: Igelströmite, Fe3+(Sb3+Pb2+)O4, and manganoschafarzikite, Mn2+SbO4, two new members of the newly established minium group, from the Långban Mn–Fe deposit, Värmland, Sweden, Eur. J. Mineral., 36, 311–322, https://doi.org/10.5194/ejm-36-311-2024, 2024.
Jonsson, E.: Fissure-hosted mineral formation and metallogenesis in the Långban Fe-Mn-(Ba-As-Pb-Sb…) deposit, Sweden, Medd. Stockh. Univers. Inst. Geol. Geok., 318, 1–110, 2004.
Jonsson, E., Hålenius, U., Majka, J., and Bosi, F.: Skogbyite, IMA 2023-085, in: CNMNC Newsletter 77, Eur. J. Mineral., 36, https://doi.org/10.5194/ejm-36-165-2024, 2024.
Jonsson, E. and Broman, C.: Fluid inclusions in late-stage Pb-Mn-As-Sb mineral assemblages in the Långban deposit, Bergslagen, Sweden, Can. Mineral., 40, 47–65, https://doi.org/10.2113/gscanmin.40.1.47, 2002.
Jonsson, E. and Billström, K.: Lead isotope systematics in the Långban deposit and adjacent sulphide mineralisations in western Bergslagen, Sweden, GFF, 131, 215–227, https://doi.org/10.1080/11035890903189751, 2009.
Lafuente, B., Downs, R. T., Yang, H., and Stone, N.: The power of databases: the RRUFF project, in: Highlights in Mineralogical Crystallography, edited by: Armbruster, T. and Danisi, R. M., Berlin, Germany, Walter De Gruyter, 1–30, https://doi.org/10.1515/9783110417104-003, 2015.
Langhof, J. and Österberg, T.: Långban's mining history, in: Långban. The mine, its minerals, geology and explorers, edited by: Holtstam, D. and Langhof, J., Raster Förlag and the Swedish Museum of Natural History, Stockholm, 51–63, ISBN 91-87214-881, 1999.
Momma, K. and Izumi F.: VESTA 3 for three-dimensional visualization of crystal, volumetric and morphology data, J. Appl. Crystallogr., 44, 1272–1276, https://doi.org/10.1107/S0021889811038970, 2011.
Moore, P. B.: Mineralogy & chemistry of Långban-type deposits in Bergslagen, Sweden, Mineral. Rec., 1, 154–172, 1970.
Moore, P. B. and Araki, T.: Braunite: its structure and relationship to bixbyite and some insights on the genealogy of fluorite derivative structures, Am. Mineral, 61, 1226–1240, 1976.
Nysten, P., Holtstam, D., and Jonsson, E.: The Långban minerals, in: Långban. The mine, its minerals, geology and explorers, edited by: Holtstam, D. and Langhof, J., Raster Förlag and the Swedish Museum of Natural History, Stockholm, 89–183, ISBN 91-87214-881, 1999.
Sheldrick, G. M.: Crystal structure refinement with SHELXL, Acta Crystallogr., C71, 3–8, https://doi.org/10.1107/S2053229614024218, 2015.
Stephens, M. B., Ripa, M., Lundström, I., Persson, L., Bergman, T., Ahl, M., Wahlgren, C.-H., Persson, P. O., and Wickström, L.: Synthesis of the bedrock geology in the Bergslagen region, Fennoscandian Shield, south-central Sweden, Geological Survey of Sweden, Ba58, 259 pp., ISBN 978-91-7158-883-8, 2009.
Wright, S. E., Foley, J. A., and Hughes, J. M.: Optimization of site occupancies in minerals using quadratic programming, Am. Mineral., 85, 524–531, https://doi.org/10.2138/am-2000-0414, 2000.
- Abstract
- Introduction
- Occurrence and mineral assemblage
- Physical and optical properties
- Chemical composition
- Infrared spectroscopy
- X-ray diffraction and structure refinement
- Relations to other mineral species
- Discussion
- Data availability
- Author contributions
- Competing interests
- Disclaimer
- Special issue statement
- Acknowledgements
- Financial support
- Review statement
- References
- Supplement
- Abstract
- Introduction
- Occurrence and mineral assemblage
- Physical and optical properties
- Chemical composition
- Infrared spectroscopy
- X-ray diffraction and structure refinement
- Relations to other mineral species
- Discussion
- Data availability
- Author contributions
- Competing interests
- Disclaimer
- Special issue statement
- Acknowledgements
- Financial support
- Review statement
- References
- Supplement