the Creative Commons Attribution 4.0 License.
the Creative Commons Attribution 4.0 License.
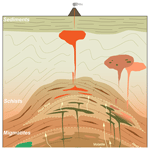
Granite magmatism and mantle filiation
Michel Pichavant
Arnaud Villaros
Julie A.-S. Michaud
Bruno Scaillet
Current granite magma generation models essentially reduce to two groups: (1) intra-crustal melting and (2) basaltic origin. A mixed, crustal, and basaltic origin and therefore a mantle filiation has been proposed for most granite magma types. In contrast, strongly peraluminous silicic magmas such as two-mica leucogranites have been classically interpreted as products of pure crustal melting. In this paper, we re-examine this interpretation and the evidence for considering leucogranites as unique among granite types. In the first part, some key aspects of the intra-crustal melting model are reviewed. Classical assumptions are discussed, such as the use of migmatites to infer granite generation processes. Our knowledge of crustal melt production is still incomplete, and fluid-present H2O-undersaturated melting should be considered in addition to mica dehydration melting reactions. The source rock remains essential as a concept despite difficulties in the identification of source lithologies from their geochemical and mineralogical signatures. Incorporating spatial and temporal variability at the source and the possibility of external inputs (fluids, magmas) would represent useful evolutions of the model. Thermal considerations bring strong constraints on the intra-crustal melting model since the absence of mafic magmas reduces possible external heat sources for melting. In the second part, the origin of a strongly peraluminous silicic volcanic suite, the Macusani Volcanics (SE Peru), is detailed. Magmas were generated in a mid-crustal anatectic zone characterized by high temperatures and heat fluxes. Crustal metamorphic rocks (metapelites) were dominant in the source region, although Ba-, Sr- and La-rich calcic plagioclase cores and some biotite and sanidine compositions point to the involvement of a mantle component. The heat necessary for melting was supplied by mafic mainly potassic–ultrapotassic magmas which also partly mixed and hybridized with the crustal melts. The Macusani Volcanics provide an example of a crustal peraluminous silicic suite generated with a contribution from the mantle in the form of mafic magmas intruded in the source region. This, as well as the limitations of the intra-crustal melting model, establishes that a mantle filiation is possible for peraluminous leucogranites as for most other crustal (S-, I- and A-type) peraluminous and metaluminous granites. This stresses the critical importance of the mantle for granite generation and opens the way for unification of granite generation processes.
- Article
(3162 KB) - Full-text XML
- BibTeX
- EndNote
The origin of granites or, more generally, of silicic magmas is a central question in Earth sciences. Historically, scientific ideas on this topic have greatly evolved with time, closely accompanying the maturation of geological concepts during the 19th and 20th centuries. The debate has become less passionate recently, but the origin of granites remains in the forefront of geological problems, being intimately linked to still-unanswered questions such as the origin, timing and mechanisms of growth of Earth's continental crust.
Since the demonstration of the magmatic origin of granites in the late 1950s (Tuttle and Bowen, 1958), there has been a considerable shift in scientific interests. Modern research on the origin of granites now concentrates on essentially two models: (1) intra-crustal melting and (2) basaltic origin. Deciding between these two alternatives is critical for global issues such as the evaluation of the respective contributions of the main geochemical reservoirs to the formation of continents, understanding the origin and concentration of elements in the crust, and constraining the mantle input through geological times. These two types of models have been recently comprehensively reviewed by Moyen et al. (2021). They are taken up again, illustrated and detailed further below.
Historically, granite diversity has constituted a major difficulty for the elaboration of unifying models. Granites have various ages; they are found in all geodynamic setting; and their mineralogical, petrological and geochemical characteristics are different from each other. Therefore, some genetic models have been preferred for certain types of granites and others for other granite types. For example, the origin of strongly peraluminous silicic granites such as two-mica leucogranites has been classically explained in the frame of the intra-crustal melting model (see Nabelek, 2020, for a recent update). Since these granites apparently show no mantle input, the basaltic origin model has been generally discarded for granite generation in continental collision orogenic belts (e.g., Nabelek, 2020; Moyen et al., 2021).
Hildreth (1981) presented a fundamentally basaltic view of lithospheric magmatism. Most silicic magmas were attributed to a mixed, crust and mantle, origin. A singular status was conceded to peraluminous two-mica leucogranites; their generation as crustal magmas was interpreted as the exception proving the basaltic rule (Hildreth, 1981). However, this interpretation was based on the classical view that peraluminous two-mica leucogranites are of purely crustal origin. If a mixed origin and a mantle filiation can be demonstrated for those granites, as for most other types, then singularity is no longer necessary and can be dropped. The consequence would be important for the origin of granites, i.e., the basaltic origin could emerge as a unifying model. From our point of view, this would represent an important step forward in the maturation of scientific ideas on this emblematic geological question.
Below, the generation of strongly peraluminous silicic magmas is re-examined. The paper focuses on the evolution of ideas and concepts on crustal melting over the last few decades, mainly in the light of authors' experiences. Studies supporting recent developments are rigorously referenced, but, for historical or classical aspects, only a few representative references are used. The paper follows a double approach based on two complementary parts. The first is a critical examination of key aspects of the intra-crustal melting model such as the spatial association between migmatites and granites, the nature of melt-producing reactions and melting mechanisms, the geochemical and mineralogical fingerprinting of source rocks, and the thermal requirements for crustal melting. The second part details magma generation on the example of a strongly peraluminous silicic volcanic suite mineralogically and geochemically equivalent to two-mica leucogranites, the Macusani Volcanics (SE Peru). Similarities but also differences with the intra-crustal melting model are emphasized. What emerges in conclusion is first the necessity of an evolution of the intra-crustal melting model toward more dynamic and open-system concepts. Second, the Macusani case shows that a mantle filiation is also possible for peraluminous leucogranitic magmas. Implications for the origin of peraluminous and metaluminous crustal magmas and for granite generation models are discussed.
2.1 Intra-crustal melting
Johannes and Holtz (1996) and Moyen et al. (2021) among others give schematic representations of the intra-crustal granite generation model, and an updated version appears in Fig. 1a. Three main features of the model are worth noting. First, in our version, basaltic magmas are absent (Fig. 1a), while they are present in very small amounts in other versions of the model (Moyen et al., 2021). Therefore, granites are generated from purely crustal rocks (intra-crustal process). The implication is that the crust is the only provider of heat, volatiles, and mass necessary for magma generation and, so, closed-system recycling and differentiation processes within the crust are favored. Second, migmatitic domains form an important part of the middle-to-lower crust. The spatial association in the field between migmatites and granites suggests that granites are formed by segregation of melt from migmatites (Mehnert, 1968). Third, granite generation takes place in the middle-to-lower crust and granite emplacement takes place in the middle-to-upper crust. This leaves a granulitic lower crust with a refractory residual composition geochemically complementary to granite (Vielzeuf and Vidal, 1990). Granulites can be interpreted to support the intra-crustal melting model.
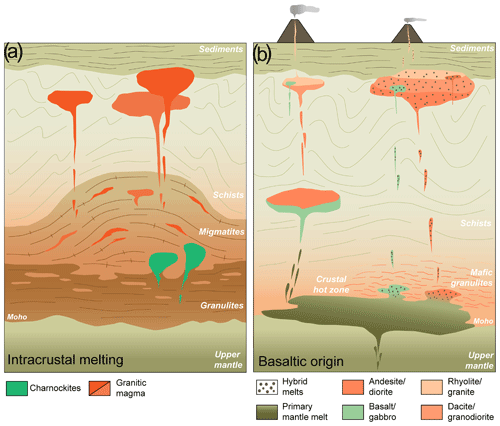
Figure 1Illustrations of the two end-member silicic magma generation models. (a) Intra-crustal melting model. Silicic magmas are generated from purely crustal rocks (intra-crustal process) in middle-to-lower crustal source regions corresponding to migmatitic domains. Basaltic magmas are absent, and silicic magmas are emplaced in the middle-to-upper crust. This leaves a lower granulitic crust with a refractory residual composition geochemically complementary to granite. (b) Basaltic origin model. On the left, closed-system fractionation of primary basaltic magma from the mantle produces the typical basalt–andesite–dacite–rhyolite arc calcalkaline sequence. On the right, extensive interaction is developed between primary basaltic magma and crustal rocks. This produces a range of hybrid magmas by open-system MASH-type processes plus refractory lower crustal lithologies including cumulates, gabbros, troctolites, mafic granulites and norites. The produced hybrid melts ascend to shallow crustal levels and contribute to the growth of granitic plutons or to the feeding of subvolcanic magma reservoirs. See text.
Several silicic magma types have been proposed to be generated in the frame of the intra-crustal melting model. The most typical are the strongly peraluminous and felsic two-mica leucogranites, equally designated as MPG granites by Barbarin (1999), that are particularly well exposed in the Variscan or Himalayan belts (Le Fort et al., 1987; Nabelek, 2020). In the field, those granites show close spatial associations with migmatitic domains (Le Fort, 1981). Mafic rocks, if present, occur only in minor amounts. Surmicaceous enclaves are typical, and mafic microgranular enclaves are generally absent (Montel et al., 1991). Leucogranite whole rocks match experimental partial melt compositions from a range of metasedimentary crustal protoliths (Patiño Douce and Harris, 1998; Castro et al., 1999; Michaud et al., 2021). Typical AFM minerals (Clarke, 1981) include muscovite, biotite, aluminum silicates, and tourmaline and, less frequently, cordierite, hercynitic spinel, and Fe–Mg garnet, a phase assemblage consistent with an origin from strongly aluminous metasedimentary sources (e.g., Michaud et al., 2021). Leucogranites have specific trace element signatures, and their isotopic compositions are typically crustal (Harris and Inger, 1992; Nabelek et al., 2020). They are chemically evolved as a consequence of element fractionation during partial melting and of possible source rock geochemical specialization and are associated with small volumes of highly differentiated products (rare-metal granites and pegmatites, Linnen and Cuney, 2005; Michaud and Pichavant, 2020; Romer and Pichavant, 2021; Pichavant, 2022). Last, strongly peraluminous granite magmas mostly crystallize to form plutons, and only some erupt as rhyolites (Raimbault and Burnol, 1998; Wang et al., 2012).
There are other granite types thought to be generated by intra-crustal melting, in particular the strongly peraluminous S-type granites from the Lachlan Fold Belt (Chappell and White, 1974). Proper S-type magmas are consistently more mafic than peraluminous leucogranites (i.e., higher FeOt, MgO and CaO, Clemens and Wall, 1984; Pichavant et al., 1988b). They carry an AFM phase assemblage that includes biotite, cordierite, Fe–Mg garnet and orthopyroxene, whereas muscovite and Al silicates are absent or rare, suggesting higher magmatic temperatures and metasedimentary protoliths less mature than for leucogranites (i.e., metagreywackes vs. metapelites, Pichavant et al., 1988b). The Variscan strongly peraluminous biotite–cordierite monzogranites and granodiorites (CPG, Barbarin, 1999) are geochemically close to the Lachlan S types (Castro et al., 1999; Moyen et al., 2017). Other granite types classically considered to be products of intra-crustal melting include the weakly peraluminous to metaluminous I- and A-type granites. Both differ from peraluminous leucogranites and S-type granites in being generated dominantly from metaigneous rather than metasedimentary sources (Chappell and White, 1974; Collins et al., 1982; Creaser et al., 1991; Dall'Agnol et al., 1999).
2.2 Basaltic origin
Several illustrations of this model have appeared in the literature following Hildreth (1981). Its key aspect is the essential role attributed to partial melting of the mantle and the generation of mantle melts, as well as to their subsequent evolution within the crust (Moyen et al., 2021). Addition of juvenile mantle magmas leads to crustal growth (e.g., Moyen et al., 2017; Gomez-Frutos et al., 2023). Below, these magmas will be designated uniformly as basaltic or mafic, although we acknowledge that a wide range of melt chemistries can be produced from the mantle (e.g., Dasgupta et al., 2010), including distinctive (potassic and ultrapotassic) compositions if magmas are generated from contaminated or metasomatized sources (e.g., sanukitoids, Castro, 2020; Gomez-Frutos and Castro, 2022; vaugnerites, Moyen et al., 2017; Bea et al., 2021; durbachites, Janoušek et al., 2020).
Two types of evolutions are distinguished as they lead to very different crustal processes (Fig. 1b). In the case of a minimal interaction with crustal rocks, cooling and fractionation of basaltic magmas produce progressively more evolved residual liquids. In detail, compositions of melts from the crystallization of basaltic magmas depend on several variables such as pressure, fO2 and the melt H2O concentration. In particular, the extent of fractionation and the amount of silicic liquids that can be generated strongly increase with the H2O content of the mantle melt (Gill, 1981). Fractionation of primitive hydrous arc magmas can generate the typical basalt–andesite–dacite–rhyolite calcalkaline sequence (Pichavant and Macdonald, 2007; Marxer et al., 2022). However, the volume of silicic magmas that can be produced by basalt fractionation is limited (∼80 % crystallization is required to produce an andesitic melt from a primary arc basalt). In addition, basaltic arc magmas may develop extensive interactions with crustal rocks (e.g., Best et al., 2016). This produces a range of intermediate to silicic magmas by open-system processes such as mixing, assimilation, hybridization, storage and homogenization (MASH, Hildreth and Moorbath, 1988). In detail, the melt compositions reflect the thermal conditions in these crustal hot zones (Annen et al., 2006) but also the nature of mantle magmas and crustal components involved and the open-system processes at work (Sinigoi et al., 2016). Crystallization of basaltic magmas coupled with reactive assimilation of crustal rocks produces a range of refractory mafic lithologies including gabbros, troctolites, norites and granulites (Bowen, 1922; Patiño Douce, 1995; Castro et al., 1999; Walker et al., 2015). These hybrid lithologies can be subsequently remobilized to yield a range of anatectic magmas of mafic lineage. Melts from MASH zones, whether differentiated or anatectic, ascend to shallow crustal levels and contribute to the growth of granitic plutons or to the feeding of subvolcanic magma reservoirs.
Silicic magmas s.l. generated with the basaltic model account for the larger part of granite and crust production. They are at the origin of most igneous rocks in arc (Cordilleran batholiths, Hildreth, 1981; ignimbrite flare-ups, Best et al., 2016) and post-collisional (Caledonian batholiths, Castro, 2020; Gomez-Frutos et al., 2023) settings. Typical silicic magmas generated with the basaltic origin model show close associations with mafic-intermediate lithologies. This goes from zoned plutons (Barbey et al., 2001; Burgess and Miller, 2008) and heterogeneous eruptive sequences (Eichelberger et al., 2000) at large scales to mafic microgranular enclaves (Bacon, 1986; Barbarin and Didier, 1992) and mafic or hybrid xenocrysts (Eichelberger, 1978) at smaller scales. Since heat is profuse in crustal hot zones, the magmas are generally hot and H2O-undersaturated, allowing them to commonly erupt. They carry a mixed isotopic signature reflecting the proportions and nature of the mantellic and crustal source components (Albarède et al., 1980; Juteau et al., 1986). Chemical variability in major and trace elements is usually strongly marked (Barbey et al., 2001; Fowler et al., 2001; Eichelberger et al., 2006; Burgess and Miller, 2008) and attributed by some to differentiation in the middle and lower crust rather than shallow-level fractionation (Gray et al., 2008; Annen et al., 2015). Granitic magmas generated with the basaltic origin model have a capacity for assimilation, not only at the source level but also during ascent and emplacement. They can acquire a superficial crustal signature (i.e., become peraluminous) as a result of assimilation of pelitic material (Erdmann et al., 2009; Clarke, 2019). Such peraluminous magmas are “made” rather than “born”, in contrast to those generated by intra-crustal melting. Hildreth (1981) suggested that S-type and other peraluminous plutons grade down to metaluminous parents.
2.3 The mantle filiation and the relative importance of crust and mantle in silicic magma generation
Silicic magmas generated with the basaltic origin model have an obvious filiation with the mantle since their origin can be traced back to mantle melting, mafic magma differentiation, assimilation and hybridization, and remobilization of hybrid protoliths. The mantle contribution is exclusive for plagiogranites and major for anorogenic peralkaline granites (Barbarin, 1999; Bonin, 2004). But it is important to stress that a mantle filiation in fact also exists for crustal silicic magmas. Heat and mass supply are interrelated, and mantle-derived magmas can trigger crustal melting when contributing to the thermal and geochemical budgets (in particular concerning volatiles) of crustal source regions (Bergantz and Dawes, 1994; Dufek and Bergantz, 2005; Manning and Aranovich, 2014; Newton, 2020). For example, the S-type granites were initially considered products of pure crustal melting (Chappell and White, 1974), but later studies identified a mantle component in their source, in the form of mantle-derived basaltic intrusions (Collins, 1996; Sandeman and Clark, 2003). The Variscan peraluminous biotite–cordierite granites to granodiorites have been interpreted as products of reactive assimilation of crustal rocks by mantle magmas (Castro et al., 1999). In the same way, the dual origin of I-type granites is now accepted (Chappell and Stephens, 1988; Castro, 2020). Some are thought to be directly derived from mantle magmas in arc settings, which confers them an obvious mantle filiation (Fig. 1b). For the others, an origin by melting of lower crustal rocks triggered by intrusion of mantle-derived magmas (sanukitoids) has been proposed (Castro, 2020), which also implies a strong participation of the mantle to magma genesis. The late-collisional calcalkaline Variscan granites (KCG, Barbarin, 1999) are interpreted to derive from potassic and magnesian mafic magmas (vaugnerites) formed by partial melting of a mantle contaminated by the regional crust (Couzinié et al., 2016; Moyen et al., 2017). Last, models for the origin of A-type granites and rhyolites invoke the presence of a juvenile mantle component in their source (e.g., Christiansen et al., 2007; Christiansen and McCurry, 2008). It is important to stress that, in the examples above, a mantle filiation is demonstrated in the form of mantle-derived magmas intruded in the source, yet the crust remains the most important (although variable) supplier of mass to the formed magmas, and, so, crustal melting is the main mechanism. Unlike most granites, peraluminous felsic leucogranites (MPG) appear to be one of the only crustal granite types where no mantle filiation has been recognized so far. Therefore, their study can serve to test whether pure crustal melting (Fig. 1a) is actually possible and realistic. This is the main issue addressed in this paper, which touches on the broader question of the relative importance of crust and mantle in crustal growth (e.g., Couzinié et al., 2016; Moyen et al., 2017; Gomez-Frutos et al., 2023).
3.1 The link between migmatites and granites
The occurrence of migmatites and the spatial association in the field between migmatites and granites constitutes one critical building block of the intra-crustal melting model (Moyen et al., 2021). The underlying assumption is that migmatitic rocks are direct witnesses of processes of granite magma generation. One place on Earth to expose granites and their postulated migmatitic source terranes in the same crustal section is the Himalayas. The two leucogranite belts parallel to the chain are in close spatial association with high-grade metamorphic rocks which have been generally assumed to represent the source of the leucogranites (Le Fort, 1975). They include migmatites, with both concordant lit-par-lit and injected leucosomes, in close spatial association with leucogranites, although the main leucogranitic masses tend to collect at the highest structural levels. Migmatites and leucogranites are broadly coeval. Ages span a range of several million years that reflect a long polyphase history for both rock types (e.g., Wang et al., 2015), consistent with migmatite–granite mutual temporal relationships being locally complex in the field. Mineralogical studies reveal systematic differences in the chemistry of major phases (plagioclase, biotite, garnet and tourmaline) between migmatites and adjacent leucogranites (Barbey et al., 1996; Yang et al., 2019). Plagioclases in leucosomes are more An-rich than in granites, and biotites have lower in the former than in the latter. Mineral compositions in migmatites also vary with the local lithology (e.g., metapelites and metagreywackes vs. orthogneiss, Barbey et al., 1996), whereas they are very homogeneous in leucogranites. Many leucosomes in migmatites are tonalitic as is also the case for inclusions in garnet (nanogranitoids, Bartoli et al., 2019). Both leucosomes and nanogranitoids commonly show positive Eu anomalies (vs. negative in the leucogranites). These results are consistent with leucosomes representing cumulate phases left after some melt escaped rather than frozen melts (e.g., Brown, 2002; Nicoli et al., 2017). Alternatively, their tonalitic compositions might reflect an early stage of H2O-saturated melting (Bartoli et al., 2019). Zircon cores in migmatites and granites have similar rare Earth element (REE) patterns, suggesting a common source. However, zircon rim REE patterns are contrasted, implying that migmatites and granites evolved under different magmatic conditions (Barbey et al., 1995). Therefore, the mineralogical and geochemical characteristics of Himalayan migmatites differ from those of leucogranites. Migmatites and leucogranites might originate from the same protoliths, but the melting processes recorded in migmatites do not match those in the leucogranite magma source region, which has been commonly assumed to reside further north and deeper than the presently exposed level (Guillot and Le Fort, 1995; Wu et al., 2020).
Our conclusion from the Himalayan case is that migmatites do not directly inform on leucogranite magma generation. The exposed migmatites are probably spatially disconnected from the leucogranite source region; hence, melting processes were different in the two sites. Migmatites should also be viewed as the end result of a long metamorphic history rather than as snapshots of anatectic melt generation. The Himalayan migmatites resided for at least several million years under P–T conditions, allowing partial melting (e.g., Harris et al., 2000; Wang et al., 2015). In comparison, timescales for melt extraction are much shorter. Melt migration via compaction operates on timescales as short as 105–106 years, and shear-assisted segregation and extraction of magma batches that feed the leucogranite laccoliths require 104–105 years, with recurrence times in the same range (Harris et al., 2000; Scaillet and Searle, 2006). Identifying such short episodes of leucogranite melt production in the long history of Himalayan migmatites is probably very difficult. The migmatite–granite connection should be recognized as definitively ambiguous (Brown, 2013; Aranovich et al., 2014).
3.2 Melt-producing reactions and melting mechanisms
Studies of migmatites and experimental simulations have stressed the diversity of melt-producing reactions in the continental crust. All involve H2O as an essential component since dry melting of quartz- and feldspar-bearing rocks requires temperatures higher than can be generally attained in the crust, recorded only in ultra-high temperature metamorphism (Clark et al., 2011). Conversely, if H2O is available, melting of most crustal lithologies becomes possible under a wide range of P and T conditions. However, silicic magmas are as a rule H2O-undersaturated. Whether for magmas feeding plutons or crystallizing in subvolcanic reservoirs, melt H2O contents are variable but less than required for H2O saturation (Scaillet et al., 1998). In other words, aH2Ocryst (the subscript refers to magma crystallization) is always <1 during most of the crystallization history. Under these conditions, a vapor phase can be present because natural igneous fluids contain volatile components with low melt solubilities such as CO2 (Holloway, 1976). H2O-undersaturated conditions at the emplacement or crystallization level necessarily imply more strongly H2O-undersaturated conditions at the source level because, in P–T space, (i) constant aH2O solidus curves in the haplogranite system have negative slopes (except for very low aH2O; see Johannes and Holtz, 1996) and (ii) magma ascent trajectories under adiabatic conditions have slightly positive slopes. Therefore, aH2Osource (the subscript refers to magma generation) is generally less than aH2Ocryst, and, so, most granitic magmas must be generated under H2O-undersaturated conditions. It follows that the only relevant melting mechanisms to be considered are those able to fulfill this constraint.
Before examining the candidate mechanisms, it is useful to list the various H2O reservoirs in crustal metamorphic rocks. They include interstitial H2O (trapped in the porosity and at grain boundaries), nominally anhydrous minerals (quartz, feldspars), hydrate phases (micas, amphibole) and an external fluid phase, assuming that fluid advection to the source region is physically possible (see below). Interstitial H2O is not negligible, and in fact 0.1 wt % interstitial H2O, which is typical for common metamorphic rocks above 400 ∘C (Yardley, 2009), is enough to generate ∼ 5.5 vol % granitic melt at 800 ∘C and 500 MPa (Clemens and Vielzeuf, 1987; Michaud et al., 2021), assuming that this type of H2O is not lost during the prograde metamorphic evolution.
The most popular model for the generation of H2O-undersaturated granitic magmas is dehydration melting (DM). Fluid-absent breakdown of hydrate phases (muscovite, biotite, amphibole) produces a H2O-undersaturated melt plus peritectic phases (Eggler, 1973; Thompson, 1982). Experimental calibrations of DM reactions show that, for muscovite, the reaction is initiated around 750 ∘C for a pressure of 8 kbar (Patiño-Douce and Harris, 1998), which are conditions compatible with those recorded in gneisses and migmatites from the Tibetan Slab in the Himalayas. The generated granitic melt contains ∼ 7 wt % H2O (corresponding to a calculated aH2O of ∼ 0.55) and therefore is H2O-undersaturated (aH2Osource < 1). Biotite DM takes place at temperatures 50–100 ∘C higher than muscovite DM and under more strongly H2O-undersaturated conditions. Melt generated by DM reactions has compositions of crustal granites (for example, melts produced by muscovite DM are leucogranitic, Patiño-Douce and Harris, 1998; Castro et al., 1999; Michaud et al., 2021). Experimental peritectic phases are identical to those in high-grade metapelitic rocks and to phases crystallizing early in crustal granites (for example, muscovite DM produces peritectic biotite, sillimanite, ilmenite and hercynite, Michaud et al., 2021). Therefore, DM has been often considered the only crustal melting mechanism. However, despite its attractiveness, the DM model is not without difficulties. Granite crystallization sequences are not always consistent with an origin by DM for the magmas. For example, Himalayan leucogranites do not have K-feldspar as the liquidus phase (Scaillet et al., 1995) as would be expected if they are generated by muscovite DM (e.g., Michaud et al., 2021; Fig. 2 and see below). Apart from considering that the Himalayan leucogranites are fractionation products (which has been proposed but relatively rarely, Scaillet et al., 1990; Wu et al., 2020), the most likely explanation of this observation is that DM is not the only melting mechanism involved in the origin of leucogranites.
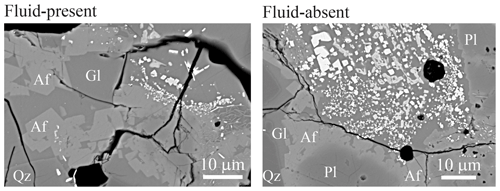
Figure 2SEM images of experimental products. Left: fluid-present charge O-3 (XH2O fluid = 0.4, Table 1) showing residual quartz (Qz) and peritectic K-feldspar (Af) coexisting with melt (Gl). Muscovite reaction products (light grey to white phases in the upper right corner) include a few residual muscovite grains (anhedral), peritectic biotite (euhedral), and smaller (≤1 µm) ilmenite and hercynite. The small grey needle-shaped phases on the right are aluminum silicates. Right: fluid-absent O-4 charge (Table 1) showing residual quartz and plagioclase and peritectic K-feldspar (Af) coexisting with melt (Gl). The extensively reacted muscovite ghost includes residual anhedral muscovite, peritectic biotite, ilmenite, hercynite and sulfide. Notice the euhedral character of Af in both charges, irrespective of the fluid regime and the continuous Af rim on residual Pl (right image).
More general problems with DM have also been identified in the literature. Volumes of granitic magmas are limited by the H2O available in the source, and the large volume of post-collisional granite batholiths requires amounts of H2O higher than can be mobilized by DM of a typical biotite–amphibole gneiss (Aranovich et al., 2014). Last, fluid-absent melting depletes the source region in H2O and other melt-compatible major and trace elements, a process consistent with a residual refractory granulitic lower crust (Vielzeuf and Vidal, 1990). However, granulites have been alternatively interpreted as products of metamorphic reactions driven by deep crustal CO2- and alkali-chloride-bearing fluids (e.g., Touret, 1971; Touret and Huizenga, 2012; Newton, 2020). In the same way, U, Rb, Cs, Li, Sn, F and Cl depletion in granulites has been attributed to transport by carbonic fluids (Cuney and Barbey, 2014). Therefore, granulites should not be considered necessarily as the signature of DM reactions.
The main granite generation mechanism alternative to DM is fluid-present melting (FPM). Although present for long in the literature (Le Fort, 1981; Montel et al., 1986), FPM has been recently re-introduced as fluid-fluxed melting (Weinberg and Hasalova, 2015). However, this designation has led to some confusion, as fluid-fluxed melting is often mistakenly assumed to involve pure H2O fluids (i.e., fluid-fluxed melting = H2O-saturated melting), in contrast with the H2O-undersaturated nature of granitic magmas emphasized above. Therefore, only H2O-undersaturated (aH2O < 1) FPM should be considered (Montel et al., 1986). In other words, crustal melting under fluid-present conditions is a viable mechanism but only with mixed fluids that reduce aH2O to <1. This is the case, for example, of fluids generated from the devolatilization of C- and S-bearing metamorphic rocks (Connolly and Cesare, 1993) or of fluids exsolved from mafic magmas (e.g., Pichavant et al., 2009; Newton, 2020). Carbonic fluids have been known for long to be involved in lower crustal metamorphism (Touret, 1971), and, in fact, mixed fluids with aH2O < 1 are most probably the rule rather than the exception in the middle-to-lower continental crust. Representative examples of crustal sections such as the Ivrea Zone demonstrate that C–H–O–N fluids coexist with granitic melts in deep anatectic domains (Carvalho et al., 2019). Variations of metamorphic fluid compositions with depth define a granite magma generation window at mid-crustal levels (Montel et al., 1986; Newton, 2020). However, FPM continues to be little considered as a melt-producing mechanism, a situation that reflects the persisting debate on the fluid regime during crustal anatexis (see Carvalho et al., 2019, for details and references). One difficulty with FPM concerns the access of fluids to the source region given the physical constraints (low porosity and permeability) in high-grade metamorphic rocks. This is usually solved by invoking deformation-assisted fluid focusing and a pulsatory influx of fluids. Shear zones tap a source of fluids (such as underplated basalt) and provide a channel into a magma source region (Weinberg and Hasalova, 2015). Another difficulty is that the FPM model requires information on the fluid composition, a difficult task given the elusive, complex and multicomponent nature of deep metamorphic fluids (e.g., Newton, 2020). Only a few experimental melting studies have been performed in the presence of fluid phases of presumed lower crustal compositions. Solidus data exist for simple granitic systems in the presence of H2O–CO2 mixtures (see Johannes and Holtz, 1996) and H2O–alkali chlorides fluids (Aranovich et al., 2013), but fluid-present melting phase equilibria and fluid–melt partitioning as a function of fluid chemistry, P and T still await systematic experimental calibrations for crustal protoliths (Conrad et al., 1988, and see below).
3.3 Trace element constraints on melting mechanisms
Trace elements are frequently used to distinguish between fluid regimes during granite magma generation. Muscovite DM produces peritectic K-feldspar (Fig. 2), whereas FPM consumes feldspars, thus imparting very different trace element characteristics to the melt (Harris and Inger, 1992). This approach has been used to discriminate between an origin by either muscovite DM or FPM for Himalayan leucogranites. Results have generally confirmed the prime role of DM, although, in some cases, both mechanisms were found to coexist (Gao et al., 2017). However, it is important to note that the two models (Harris and Inger, 1992) are associated with strongly contrasted melt fractions (14 % for DM and 40 % for FPM), and, so, comparison between trace element signatures is not made at constant melt fraction. The 40 % melt fraction in the FPM case also seems very elevated. It is the consequence of assuming aH2O = 1 (H2O saturation) during FPM melting (Harris and Inger, 1992), an unrealistic situation for granite magma generation as emphasized above.
Table 1Conditions and results of the melting experiments on DRO09 orthogneiss.

All experiments performed at 800 ∘C and 4 kbar for 2 weeks in an internally heated vessel pressurized with Ar–H2 gas mixtures. See Michaud et al. (2021) for details. a Muscovite seeded. b Mass fraction of fluid in the charge (wt %); 0 indicates fluid-absent conditions. XH2O = initial molar in the fluid; 0 indicates fluid-absent conditions. log fO2 and ΔNNO determined from Co–Pd sensors (Michaud et al., 2021). H2O in glass estimated with the by-difference method. c Phase proportions in wt % determined by mass balance from electron microprobe compositions. Abbreviations: silicate melt (L), quartz (Qz), K-feldspar (Af), plagioclase (Plag), biotite (Biot), orthopyroxene (Opx), garnet (Gt), sillimanite (Sill), ilmenite (Ilm) and apatite (Ap).
One important point to be noted is that the stoichiometry of FPM reactions varies with aH2O. FPM experiments of a muscovite-rich orthogneiss (Table 1) show that K-feldspar is consumed at high aH2O (H2O–CO2 fluids with initial XH2O = 1, 0.8), consistent with the modeling (Harris and Inger, 1992). Yet, it becomes a peritectic phase at low aH2O (H2O–CO2 fluid with initial XH2O = 0.4; Fig. 2). Melts formed under such low aH2O are enriched (high , low Ba) since K-feldspar is part of the peritectic assemblage and their trace element compositions are similar to melts produced by muscovite DM (Table 2; Fig. 3). In comparison, melts generated under high aH2O have low and high Ba (Table 2; Fig. 3). These results show that the generation of melts with enriched trace element signatures is not restricted to DM. More generally, a precise knowledge of the melting reaction (nature and proportions of peritectic phases, melt fractions) is a pre-requisite for modeling the trace element behavior (Michaud et al., 2021).
Table 2Major and trace element compositions of experimental glasses.
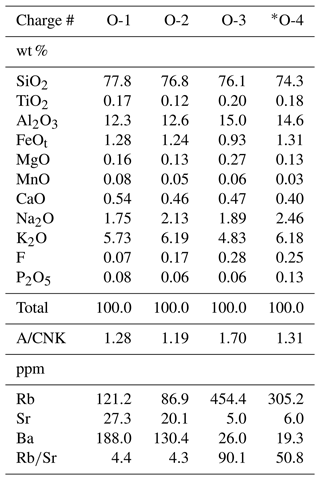
Major elements analyzed by electron microprobe. Trace elements analyzed by laser ablation inductively coupled plasma mass spectrometry (LA-ICP-MS). See Michaud et al. (2021) and Pichavant et al. (2023) for details on the methods. * Muscovite seeded.
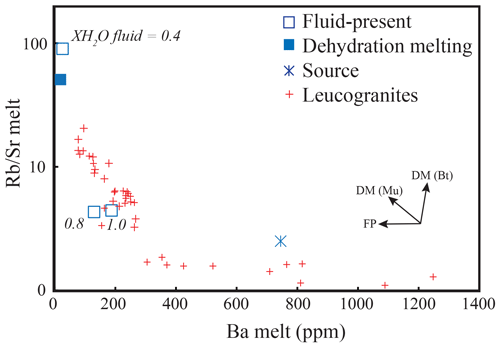
Figure 3Ba concentrations and of experimental melts produced by fluid-present (open squares) and muscovite dehydration melting (solid square) of a muscovite-rich orthogneiss (star). Experimental conditions are given in Table 1, and melt concentrations are from Table 2. The fluid-present experiments are labeled with XH2O fluid (1.0, 0.8, 0.4, Table 1). Notice the contrast in melt compositions between the XH2O fluid = 1.0 and 0.8, on the one hand, and XH2O fluid = 0.4, on the other hand, a consequence of the presence of peritectic K-feldspar in the latter charge (Fig. 2). Trace element melt compositions in the dehydration melting charge are similar to those in the XH2O fluid = 0.4 charge. Whole-rock compositions of Himalayan leucogranites are plotted for comparison (crosses; data from Le Fort, 1981; Scaillet et al., 1990; Inger and Harris, 1993; Gao et al., 2017). The arrows show partial melting vectors for fluid-present melting (FPM), dehydration melting of muscovite (DM (Mu)) and dehydration melting of biotite (DM (Bt)) after Inger and Harris (1993).
In conclusion about melting mechanisms, it is important to stress that DM and FPM are not exclusive from each other and should be rather viewed as complementary (Carvalho et al., 2019; Michaud et al., 2021). However, accepting FPM as a viable mechanism would fundamentally shift the intra-crustal melting paradigm from a closed to open system since an external fluid is involved in the case of fluid-present melting.
3.4 The source rock concept from a geochemical viewpoint
The source rock concept is a fundamental component of the intra-crustal melting model. It has proven its critical importance and at the same time its practical utility with the emergence of global granite classifications (Chappell and White, 1974). The concept has gained wide acceptance despite obvious difficulties, with the most critical being that granite source regions are not always accessible to direct observation as is the case, for example, for most Variscan granites. Additionally, migmatites may not directly inform on granite generation, and all granulites are not necessarily products of DM reactions. Source-granite genetic relationships are also challenging to establish (e.g., Wolfram et al., 2017), and, in most cases, they are limited to demonstrating identical ages for granite and high-grade metamorphism recorded in candidate source rocks (e.g., Gebelin et al., 2009).
Geochemical, mainly isotopic, data on granites have been commonly used to constrain the nature of their source rocks. For this inverse approach to be valid, the isotopic composition of the melt should be identical to that of its source, an assumption which has been tested in a few rare cases. For example, Sri in Himalayan leucogranites and Tibetan Slab metamorphic rocks overlap. Thus, the Tibetan Slab could represent the protolith involved in the generation of leucogranites. However, both leucogranites and metamorphic rocks show a wide range of Sri, respectively from 0.73 to 0.77 and from <0.73 to 0.78 (Guillot and Le Fort, 1995), which raises several issues. First, the very large ranges in Sri do not convincingly demonstrate an affiliation of the metamorphic rocks to the leucogranites. Second, the Sri heterogeneities, both for leucogranites and metamorphic rocks, need an explanation. The interpretation proposed is that leucogranites inherit their variable Sri from isotopically heterogeneous source rocks (Guillot and Le Fort, 1995). However, this pushes back the problem of knowing why those metamorphic rocks are heterogeneous in Sr isotopic composition. The lithological heterogeneities of crustal protoliths at the meter scale and the possibility that metamorphism and anatexis do not fully homogenize seriously complicate the interpretation of Sr isotopic compositions of crustal granites.
The melting behavior of mineral assemblages isotopically heterogeneous in Sr has been experimentally investigated using a simple protolith made of plagioclase and biotite (Hammouda et al., 1996). The generated melts have ratios that reflect the proportion of plagioclase and biotite consumed in the melting reaction. Melt ratios vary with time due to progressive changes in the stoichiometry of the melting reaction that reflects the contrast in melting kinetics between plagioclase and biotite. They are different from the bulk of the starting mineral mixture (the source), although melts in long experiments tend to approach it. Thus, the Sr isotopic signature of the formed melt is primarily controlled by melting mechanisms and kinetics rather than by the composition of the source. Mineral-scale isotopic heterogeneities are not restricted to the source region and are also found at the magma emplacement level. Granitic rocks in the Elba island show variability between minerals, matrices and whole rocks (Farina et al., 2014). Such large heterogeneities (Sri ∼ 0.730 for biotite vs. 0.715–0.720 in K-feldspar megacrysts from the Mount Capanne pluton) have long been interpreted in terms of variable contributions of isotopically contrasted components (e.g., crust and mantle) to magma genesis. However, this traditional view is now being challenged, and the interpretation proposed for the Elba island granites postulates mixing, in the magma reservoir, of several individual magma batches formed by disequilibrium melting of the same source rocks (Farina et al., 2014).
Recent studies on migmatites have emphasized the complex behavior of not only the Sr but also the Nd isotopic system. Sr–Nd isotopic data for leucosome and restite pairs from Variscan migmatites show that the low-T rocks are near equilibrium, whereas most pairs from the high-T rocks record fractionation of Sr and Nd isotopes between leucosome and restite (Wolf et al., 2019). Explanation of these results involves a combination of factors at the mineral scale. Radiogenic and unradiogenic minerals that contribute in different proportions to leucosome and restite can have different isotopic ratios (variable protolith ages) and variably equilibrate during the metamorphic history. The key point is that neither the leucosome nor the restite inherits its radiogenic isotopic signature from the bulk source in a simple way.
Results for the Nd but also for the Pb and Hf isotopic systems demonstrate that the isotopic compositions of anatectic melts are controlled by the behavior of accessory phases, in particular zircon, monazite and apatite. For Pb, Hogan and Sinha (1991) modeled the influence of melting of accessory zircon and monazite on Pb isotopic compositions. Two cases were distinguished: granites with near-homogeneous and with heterogeneous Pb isotopic signatures. In the former, which corresponds to leucogranites (e.g., Manaslu leucogranite, Hogan and Sinha, 1991), Pb isotopic compositions are controlled by contributions from major mineral phases in the source (mainly feldspars) because solubilities of zircon and monazite in the melt are limited at low temperatures. Conversely, if zircon and monazite can dissolve in significant amounts because of higher temperatures in the source, the Pb isotopic signature of the melt becomes variable, reflecting the amount and the isotopic composition of zircon contributing to the melting reaction. In the same way, kinetic models of dissolution of apatite, zircon and monazite in anatectic melts have stressed the possibility to generate a range of melt Nd and Hf isotopic signatures from the same source (Zeng et al., 2005; Tang et al., 2014). Therefore, the conclusion that emerges is that Sr, Nd, Pb and Hf isotopes are more indicators of mechanisms, both at the source (melting reactions and rates, mineral phases involved) and at the pluton assembly level (mixing between individual magma batches), than of source lithologies and components.
In comparison, oxygen isotopes provide a more global image of the source since oxygen is a major element in most phases of a silicic magma. Leucogranite whole-rock and mineral separate data have generally yielded high δ18O values mostly between 11 ‰ and 14 ‰ (see Nabelek, 2020, for a review). Connections between leucogranites and potential source rocks have been examined for the Himalayan, Variscan and other representative examples such as the Black Hills (USA). Almost invariably, the δ18O values of leucogranites are in the same range as the local metapelites and metagreywackes (France-Lanord et al., 1988; Scaillet et al., 1990; Nabelek et al., 1992). The high δ18O values of strongly peraluminous leucogranites point to protoliths with a component having gone through the weathering cycle and/or partially equilibrated with seawater (see Nabelek, 2020). However, this includes a wide range of potential sedimentary rocks from mature (shales) to immature (greywackes). Therefore, oxygen isotope data in general lack the resolution necessary for a detailed fingerprinting of magma protoliths, although, in the Black Hills, the slightly higher δ18O values in tourmaline than in two-mica leucogranites have been interpreted to indicate more muscovite-rich sources for the latter than the former (Nabelek, 2020). The high δ18O values imply that the metasedimentary component is dominant in the source of leucogranites but does not rule out that a metamorphosed igneous component (orthogneiss) can be present as inferred from the Sr and Nd signature of some Variscan leucogranites (Turpin et al., 1990; Michaud et al., 2021).
3.5 The source rock concept from a mineralogical viewpoint
Most silicic magmas carry materials that potentially inform about their origin – either enclaves, restitic minerals or early crystallized magmatic phases. These can provide mineralogical constraints on the nature of the protoliths. Such constraints are particularly valuable to fully exploit the information brought by granitic magmas about unexposed parts of the continental crust. Mineralogical information at the source level is also useful for practical purposes. For example, identification of mineral carrier phases is required to understand how rare elements and critical metals are incorporated in anatectic melts. The response of radiogenic isotope systems largely depends on the nature and behavior of major and accessory minerals in the source, as illustrated in the preceding section.
Enclaves are precious sources of information on the unexposed parts of silicic magmatic systems. Metapelitic enclaves of centimetric to decimetric size representing fragments from the source are relatively common in shallow-level crustal granites (Montel et al., 1986, 1991). However, the possibly needs to be considered that they represent country rocks accidentally incorporated during magma ascent (Vernon, 2007). In the South Mountain Batholith (Nova Scotia), garnet-rich segregations have been interpreted as partially assimilated metapelitic country rocks and cordierite- and biotite-rich zones as products of crystal accumulation and fractional crystallization, respectively (Erdmann et al., 2009). The dark microgranular enclaves found in many silicic-intermediate plutonic and volcanic systems represent small (most commonly decimetric) blobs of relatively mafic, high-temperature magma chilled within a cooler, more silicic host (Bacon, 1986; Barbarin and Didier, 1992). They are samples of magma that coexisted in the same igneous system as their host. In general, the nature of enclaves provides a first-order indication on the origin of the silicic magma. Mafic microgranular enclaves are generally absent in felsic peraluminous granites (but see Zheng et al., 2016; Wu et al., 2020). Their presence in silicic magmas generated with the basaltic origin model (Burgess and Miller, 2008) as well as in relatively mafic peraluminous crustal granites (Castro et al., 1999) reveals the existence of the mantle filiation.
Granites rooted in high-grade metamorphic rocks, either migmatites or granulites, commonly carry enclaves or individual phases whose origin can be tracked back to their source region (Barbero and Villaseca, 1992; Wolfram et al., 2017). In comparison, the identification of restitic or early magmatic phases in shallowly emplaced crustal silicic magmas is more difficult mainly because most re-equilibrate chemically during crystallization, especially in plutonic environments. Texturally early minerals can represent restites or, alternatively, correspond to the first phases in the magma crystallization sequence. For example, sillimanite inclusions are very abundant in most phenocrysts from the strongly peraluminous Macusani Volcanics (Pichavant et al., 1988a). On the basis of morphological and textural criteria, they have been interpreted as early magmatic rather than restitic (Pichavant et al., 1988a). Calcic plagioclase cores were initially considered restites (Chappell et al., 1987) despite their textures being typically igneous (e.g., oscillatory zoning, Pichavant et al., 1988a). They are now interpreted as early magmatic phases (Pichavant et al., 1988a, 2023; Vernon, 2007; see below). In contrast, a restitic origin can be demonstrated for garnet as in the strongly peraluminous Flagstaff Lake Complex rocks (Dorais and Campbell, 2022). This short survey illustrates the ambiguities in assigning a restitic, magmatic or xenocrystic origin to individual minerals in silicic magmas, in particular those crystallized as plutonic rocks. However, refractory phases such as zircon demonstrate that restites and inherited xenocrysts can make up a significant fraction of magmatic mineral assemblages.
Mechanical separation between restites and melt has been proposed to explain the compositional variability in crustal granite suites (White and Chappell, 1977; Chappell et al., 1987). Silicic magma compositions can represent melt-peritectic phase mixtures in different proportions; granite compositional variability would thus reflect variable entrainment of peritectic phases (e.g., Stevens et al., 2007; García-Arias and Stevens, 2017). The peritectic entrainment model has been widely applied to crustal granite suites. S-type granites have compositions more mafic (higher FeOtot+MgO and, to a lower extent, CaO and TiO2) than experimental partial melts from crustal protoliths (e.g., Stevens et al., 2007; García-Arias and Stevens, 2017; García-Arias, 2018). This is consistent with entrainment of peritectic phases, although mafic refractory lithologies present in the source region could also play a role (Carvalho et al., 2017). We also point out that experimental anatectic melts can be quite mafic. Examples of silicic experimental melts with FeOtot+MgO and CaO contents in the range of S-type granites exist in the literature (Gardien et al., 1995; Cadoux et al., 2014). For the melt compositions plotted in Fig. 4, the FeOtot+MgO content at equilibrium with crustal mineral phase assemblages and compositions regularly increases with the melt H2O content at fixed temperature and fO2 and with decreasing fO2 at fixed temperature. The FeOtot+MgO contents reach concentrations higher than the reference experimental melts considered by García-Arias and Stevens (2017), and it is likely that their conclusion about experimental melts being less mafic than S-type granites reflects average H2O and fO2 conditions in a particular group of experiments (Fig. 4). In addition, alternatives to the entrainment model have been proposed to explain granite geochemical variability. For example, compositions of peraluminous cordierite monzogranites from the Central Iberian Zone reflect melting and reactive assimilation of crustal rocks by mantle magmas (Castro et al., 1999). Chemical variations in several S-type granite series have been interpreted in terms of multicomponent mixing processes (Collins, 1996; Sandeman and Clark, 2004). In comparison, major element compositions of leucogranites have been adequately reproduced experimentally (Patiño-Douce and Harris, 1998; Castro et al., 1999; Michaud et al., 2021), indicating that crustal melting mainly controls of the magma chemistry.
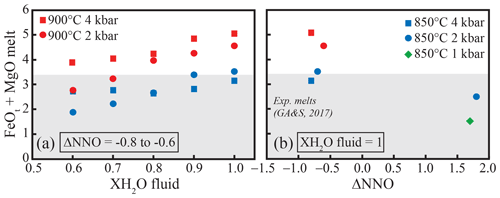
Figure 4Influence of (a) the mole fraction of H2O in the experimental fluid (XH2O fluid) and of (b) ΔNNO (deviation from the Ni–NiO oxygen buffer at the same T and P) on concentrations of FeOt+MgO in experimental silicic melts. Data from Cadoux et al. (2014). In panel (a), the ΔNNO range indicated pertains to charges with XH2O fluid = 1. Charges with XH2O fluid <1 have slightly lower ΔNNO. In panel (b), only charges with XH2O fluid = 1 are shown. Melts are rhyodacitic to rhyolitic and at equilibrium with orthopyroxene + ilmenite as Fe- and Mg-saturating phases for ΔNNO < 0 and orthopyroxene + magnetite for ΔNNO > 0. At 1 kbar, amphibole joins orthopyroxene + magnetite. The grey domain is the field of experimental partial melts from García-Arias and Stevens (2017). Melts from Cadoux et al. (2014) have FeOt+MgO concentrations (maximum 5.06 wt %) that exceed the maximum (3.41 wt %) of reference experimental melts (grey domain), especially at 900 ∘C for XH2O fluid >0.7 and at 850 ∘C for ΔNNO < 0.5. Note that melt FeOt + MgO concentrations continue to increase below ΔNNO < −1. There is no apparent necessity to limit the FeOt+MgO of crustal silicic melts to ∼ 3.5 wt % maximum (García-Arias and Stevens, 1997). Additional examples in the literature of experimental melts with FeOt+MgO > 3.5 wt % include Gardien et al. (1995).
The CaO content is another key geochemical indicator of crustal granite suites. For a given FeOtot+MgO, S-type granites have CaO mostly higher than their postulated sources and melts generated from them, with the latter calculated with thermodynamic models (García-Arias, 2018). However, melt CaO constrained from thermodynamic models is known to underestimate concentrations in partial melting experiments; CaO in experimental glasses is systematically higher than in models, with differences up to 100 % for some T-H2O conditions (Bartoli and Carvalho, 2021). As a corollary, models calculate plagioclase An contents much higher than in experiments (Bartoli and Carvalho, 2021; Pichavant et al., 2019). Although these discrepancies have been tentatively explained, for example, by assuming either disequilibrium melting or entrainment of Ca-rich plagioclase (García-Arias, 2018), they rather reflect our limited ability to calculate anatectic melt compositions with the current calibrations of thermodynamic models (Holland and Powell, 2011; White et al., 2014; Bartoli and Carvalho, 2021). In particular, experimental data on the equilibrium Na–Ca partitioning between plagioclase and hydrous silicic melt are still scarce (Pichavant et al., 2019). We therefore conclude that the interpretation of chemical variability in crustal granite suites needs reconsideration (e.g., Patiño-Douce, 1995).
Summarizing the two sections above, it is important to emphasize that the source rock concept remains critical despite difficulties in identifying source rocks through their geochemical and mineralogical signatures. However, significant evolutions in the source rock paradigm should be encouraged. The importance of melting mechanisms and kinetics is now better recognized, as seen in the interpretation of radiogenic isotope data. It is becoming accepted that a given source can yield a range of granite images depending on rates of melting mechanisms. In the same way, there is a need for the source rock concept to accommodate the possibility of external fluids and, as discussed below, of external magmas in the source region.
3.6 Thermal requirements for melting
Granite generation is a process intimately linked to the thermal evolution of the lithosphere. It requires a significant amount of heat, which in principle is supplied by crustal rocks through radiogenic heat production, conductive transfer from the underlying mantle and intrusion of hot mantle-derived magmas. In the frame of the intra-crustal melting model, mantle magmas are absent and heat sources reduce to crustal rocks and conduction from the underlying mantle. This limits the range of possibilities for silicic magma generation so that the heat requirement provides a particularly critical test of the model (Fig. 1a). Nevertheless, thermal modeling studies have established the possibility for the crust to melt without heat advection from hot mantle magmas. Thermal relaxation of a tectonically thickened crust leads to its partial melting (England and Thompson, 1986). Melting is favored in crustal rocks with high heat productivity (Jaupart and Provost, 1985; Pinet and Jaupart, 1987; Bea, 2012) and also when the heat flux from the mantle is increased, for example, following delamination of the lower crust and lithospheric mantle (Moyen, 2020). Shear heating provides an additional heat source, and thermomechanical simulations of the Himalayan collision have shown that large-scale crustal melting can take place without any heat source other than radiogenic production and shear heating (Nabelek and Nabelek, 2014). The possibility of an intra-crustal origin for the leucogranites is thus confirmed, although present-day partially molten zones in the Himalayas have a more limited extension than predicted by the models (Nabelek and Nabelek, 2014). Therefore, we conclude that the intra-crustal melting model has successfully passed the thermal modeling test but only for specific conditions of the parameters.
Are petrological characteristics of granites consistent with generation without heat advection from mantle-derived magmas? Granites such as two-mica leucogranites lack a systematic association with mafic rocks in the field, and they contain surmicaceous enclaves, although the discovery of mafic microgranular enclaves in some Himalayan leucogranites (Zheng et al., 2016; Wu et al., 2020) opens the way for alternative hypotheses (see below). Leucogranite magma generation is thought to occur mainly via muscovite DM at deep crustal levels (i.e., at ∼ 750 ∘C for 8 kbar, Patiño-Douce and Harris, 1998; Nabelek, 2020). Liquidus temperatures of leucogranitic magmas are limited to ∼ 800 ∘C (Scaillet et al., 1995). Under these P–T constraints, the need for an external heat source is less critical since melting in the middle-to-lower crust requires a minimum excess enthalpy (see Moyen, 2020). However, it is worth emphasizing that this temperature range can be attained only in specific parts of the crust and for favorable values of the model parameters (England and Thompson, 1986; Bea, 2012). For crustal granites more mafic than leucogranites such as S-type granites whose generation is thought to involve biotite DM at higher temperatures, even more extreme model parameters would be required (see Clark et al., 2011). However, for such rocks, for example, the peraluminous biotite–cordierite monzogranites of the Central Iberian Zone, mantle magmas have been attributed an important role (Castro et al., 1999). In the same way, a hybrid mantle-crust origin has been proposed for the strongly peraluminous mafic S-type monzogranites of the Cordillera Oriental of SE Peru (Sandeman and Clark, 2003). A mantle component is present in the source region of S-type granites from the Lachlan Fold Belt (Collins, 1996). Therefore, for mafic peraluminous granite types, the question is more whether pure crustal melting is actually realistic as opposed to feasible thermally.
3.7 Summary
Examination of the intra-crustal melting model has identified a number of shortcomings and stresses the need to reevaluate several key assumptions. Migmatites should not be indiscriminately viewed as images of granite magma generation sites. Dehydration melting reactions are not the only way to generate H2O-undersaturated granitic melts, and alternative mechanisms such as fluid-present (but H2O-undersaturated) melting need more consideration. The source rock remains an important concept, although defining source rock lithologies geochemically or mineralogically is difficult and often ambiguous. Last, thermal models confirm the possibility for the crust to melt without heat advection from hot mantle magmas but only under specific conditions. These criticisms challenge the intra-crustal melting model globally. They emphasize the uncertainties concerning the generation of peraluminous leucogranites as well as of other granite types with a major crustal source component, such as S-type granites.
The case example detailed below is the Miocene–Pliocene Macusani Volcanics from SE Peru. It is characterized by a volcanic mode of emplacement, which is rare although not exceptional among the strongly peraluminous silicic magma series (see below). Mineralogically and geochemically, the Macusani Volcanics are equivalents to the Variscan and Himalayan two-mica leucogranites and rare-metal granites (Pichavant et al., 1988b, 2023). Therefore, differences in magma generation mechanisms with leucogranites cannot be attributed to compositional effects.
4.1 Geological setting
The Macusani Volcanics are located in the Department of Puno, southeastern Peru, ∼ 50 km north of Lake Titicaca. The area belongs to the Cordillera de Carabaya segment of the Central Andean Eastern Cordillera (Cordillera Oriental). It exposes a diverse assemblage of (1) Oligocene to Miocene (the Picotani Group, 22–26 Ma) and (2) Miocene to Pliocene (the Quenamari Group, ∼ 4–17 Ma) volcanic and hypabyssal intrusive rocks. The Quenamari Group formations (to which the Macusani Volcanics belong) outcrop mainly in two separate volcanic fields (the Quenamari and Picotani fields) and in a few other smaller locations (see Pichavant et al., 2023, for an update and references).
In the Quenamari field, the volcanic rocks (Macusani Volcanics) cover an area of 860 km2 at an average altitude of ∼ 4400 m, plus a few hypabyssal plutonic rocks. The maximum thickness of the sequence is 500 m, and its estimated volume is 430 km3 (Cheilletz et al., 1992). The volcanic rocks unconformably overlie deformed Paleozoic sedimentary strata, Permian to Jurassic sedimentary, volcanic and plutonic rocks, and Oligocene to Miocene volcanic rocks from the Picotani Group. They consist of mainly non-welded ash-flow tuffs with a minor amount of chemically highly evolved obsidians mostly found as pebbles and more rarely as inclusions in the volcanic deposits (Pichavant et al., 1987). The obsidians have a residual major element composition, and their trace element concentrations suggest an origin by fractionation from interstitial liquids present in ash-flow tuffs (Pichavant et al., 2023). Two major eruptive cycles at 10±1 and 7±1 Ma have been recognized from data (Cheilletz et al., 1992), but volcanic activity continued until ∼ 4 Ma as the obsidians have ages (4–5 Ma) younger than the two main magmatic pulses (Pichavant et al., 2023). In the Picotani field, the volcanic rocks are older (16–18 Ma, Pichavant et al., 1988a). They comprise two main units covering a total areal extent of 160 km2 for a thickness of 200 m (magma volume of 32 km3 minimum). The volcanic rocks unconformably overlie the sedimentary basement and also the older Picotani Group rocks (see Pichavant et al., 2023).
4.2 Mineralogical and geochemical characteristics of the magmas
The Macusani ash-flow tuffs are crystal-rich (40 vol %–55 vol %), with quartz, sanidine, plagioclase, biotite, muscovite, andalusite, cordierite, tourmaline, apatite and ilmenite phenocrysts (Pichavant et al., 1988a). Accessory sillimanite, hercynitic spinel, zircon and monazite are found mainly as inclusions in other mineral phases. The phenocrysts are essentially unaltered except cordierite. They are embedded in a fine-grained matrix, mostly devitrified and altered to clay minerals. The obsidians are very crystal-poor, but they host microphenocrysts mineralogically identical to the phenocrysts in the tuffs (Pichavant et al., 1988a). In the Picotani field, quartz, sanidine, plagioclase, biotite, muscovite and apatite are the main phenocrysts, and accessory minerals include ilmenite, sillimanite, zircon and monazite (see Pichavant et al., 2023, for an update and references).
Compositions of the Macusani ash-flow tuffs are all very similar, rhyolitic, strongly peraluminous (A/CNK > 1.2, normative corundum > 2 %), and felsic with high Na2O and K2O and low FeOt, MgO, CaO and TiO2. The fluxing elements (P2O5, F, Li2O and B2O3) have concentrations at the 0.1 wt % level in the ash-flow tuffs, increasing to > 0.5 wt %–1 wt % in the obsidians. Trace element patterns are characterized by high lithophile (Be, Rb, Cs) and rare metal (Sn, W, Nb, Ta, U) and low Ba, Sr, Eu, Zr, Th and Pb concentrations. The isotopic compositions (Sri: 0.721–0.726; εNd: −8.96 to −9.35; : 18.74–19.45; : 15.66–15.72; δ18O: +12 ‰ (glasses), +11.5 ‰ to +12.7 ‰ (quartz)) are typically crustal (see Pichavant et al., 2023, for an update and references).
4.3 Magma generation
On the basis of the mineralogical, geochemical and isotopic data summarized above, an origin of Macusani magmas based on the intra-crustal melting model was initially proposed (Pichavant et al., 1988b). The presence of aluminous phases (biotite, cordierite, tourmaline, sillimanite, hercynite) in the early magmatic assemblage (interpreted as either restites or early phenocrysts) indicates a major pelitic component in the source region. This metasedimentary protolith accounts for the strongly peraluminous nature of the generated magmas. It is consistent with the presence of aluminous phenocrysts (muscovite and andalusite) in the main magmatic assemblage and with the isotopic data (see above, Pichavant et al., 1988b). The ash-flow tuffs all have felsic and strongly homogeneous major element compositions; interstitial melts and glass inclusions are uniformly rhyolitic (Pichavant et al., 2023). Thus, the possibility that the ash-flow tuffs represent fractionation products from mafic peraluminous magmas can be excluded (Pichavant et al., 1988b). Therefore, the Macusani Volcanics were interpreted as products of anatexis of purely crustal rocks. H2O for melting was supplied by the source rocks without input from external fluids (internal buffering of aH2O), and magma generation resulted essentially from muscovite DM combined with incipient biotite DM (Pichavant et al., 1988b).
Types of source rocks; nature of early mineral phases; phenocryst assemblages; and bulk rock major, trace element and isotopic compositions for the Macusani Volcanics are closely similar to those for Variscan or Himalayan leucogranites (Pichavant et al., 1988a, b; Nabelek, 2020). Temperatures (constrained from biotite melting relations, Pichavant et al., 1988a) were elevated (∼ 800 ∘C) in the magma source region, in the upper range of values inferred for leucogranite generation (Scaillet et al., 1995). Heat fluxes (i.e., amount of heat per time unit) were also particularly high as demonstrated by widespread disequilibrium melting of biotite (Pichavant et al., 1988a) and by the large amounts of sillimanite inclusions in phenocrysts, possibly indicating massive assimilation of aluminous materials at the magma production site (e.g., Noble et al., 1984; Clarke, 2019). These thermal conditions make the need for an external heat supply particularly acute, especially considering that metamorphic grades are low in the thick metasedimentary sequence that underlies the Cordillera Oriental. Additionally, the lack of Fe–Mg garnet and the presence of cordierite and hercynite as biotite breakdown products (Pichavant et al., 1988a) indicates that the anatectic zone was no deeper than the middle crust. This corresponds to P–T conditions that are very costly in terms of enthalpy to bring to the system for melting (Moyen, 2020), thus maximizing the need for an external heat source, initially attributed to mafic intrusions (Pichavant et al., 1988b).
Until recently, no mafic component had been identified in the Macusani magma source region. No mafic magma erupted contemporaneously with the silicic volcanics in the area. Mafic microgranular enclaves have not been described in Macusani ash-flow tuffs, and mineral assemblages lack mafic xenocrysts (Pichavant et al., 1988a, 2023). However, trace element analyses reveal the presence of a Sr-, Ba-, La-rich signature in plagioclase, sanidine and biotite. This enrichment is systematic in calcic plagioclase cores from the early magmatic assemblage (Pichavant et al., 1988a), being also present and sometimes even more marked in rare An ∼ 25 plagioclases intermediate between cores (An30–45) and the main phenocryst population (An10–20, Pichavant et al., 2023). Mafic magmas that erupted in the area between 21 and 25 Ma include volumetrically dominant potassic to ultrapotassic (K-UK) rocks (lamproites, minettes, kersantites) which carry a specific, LILE-enriched, F-rich and reduced signature, plus a few calcalkaline basalts (Pichavant et al., 2023, and references therein). Mafic magmatism was practically absent between 5 and 17 Ma (i.e., during eruption of the Quenamari Group). However, eruption of K-UK rocks as young as 0–2 Ma in the magmatic lineament NW of the Cordillera Oriental suggests that mafic activity in fact continued, although unexposed at the surface. By analogy with plagioclases in older (21–25 Ma) hybrid rocks, the calcic plagioclase cores in the Macusani Volcanics are interpreted as products of hybridization and mixing between K-UK and anatectic melts in the source region (Pichavant et al., 2023). The identification of a mafic component is consistent with the heat requirements emphasized above and the role attributed to mafic magmas. A low-velocity zone has been imaged by geophysical methods at mid-crustal depths beneath the Cordillera Oriental and interpreted as a partially molten layer caused by mantle magmatism and heat advection (Ma and Clayton, 2014).
The finding of a mantle component means that the Macusani magmas can no longer be considered purely crustal (Pichavant et al., 1988b). Generation of the Macusani Volcanics borrows several key aspects to the basalt origin model, such as the specific thermal regime and the presence of a mafic component in the source region. MASH-type hybridization processes, although present, are limited to the crystallization of calcic plagioclase cores and to the assimilation of aluminous crustal materials. Other aspects such as magma fractionation and volcanic emplacement also fit more in the basaltic origin than in the intra-crustal melting model. Crystallization differentiation of rhyolitic interstitial melts in ash-flow tuffs yields the highly fractionated liquids represented by the obsidians (Pichavant et al., 2023). Magma fractionation was promoted by elevated melting temperatures in the source which allowed breakdown of F-enriched micas and tourmaline and released fluxing elements (F, B) in the anatectic melts. This led to lowering of solidus temperatures and melt viscosities, thus allowing crystal fractionation to be pushed further down temperature. The volcanic emplacement reflects high temperatures in the source, being also facilitated by the mid-crustal magma production site. The high F concentrations of Macusani micas are also inherited from the source. DM conditions of F-rich micas occur at higher temperatures than for F-poor micas (Pichavant et al., 1988a). Thus, generation of H2O-undersaturated melts is promoted, and magma ascent and eruption is favored. Several characteristics of the Macusani Volcanics result from magma generation processes that are unusual in the intra-crustal melting model.
5.1 Representativity of the Macusani Volcanics
The Macusani case illustrates a mechanism of peraluminous silicic magma generation where a mantle contribution, in the form of intruded mantle-derived magmas in the source region, can be recognized. Although a mantle component is involved, the mineralogical and geochemical data demonstrate that the crustal component is largely predominant, and, so, the Macusani magmas have a definitely crustal origin.
The representativity of the Macusani volcanic suite among peraluminous silicic magmas is in no doubt (Pichavant et al., 1987, 1988a, b, 2023). For example, the Macusani obsidians are currently used as proxies for a specific type of pegmatite compositions (London, 2015). The early magmatic/restitic phases in the Macusani Volcanics are identical to assemblages found in high-grade metamorphic rocks and surmicaceous enclaves representative of leucogranite source regions (Pin and Vielzeuf, 1983; Montel et al., 1986; Barbero and Villaseca, 1992; Bea et al., 1999; Gebelin et al., 2009). The lack of Fe–Mg garnet constitutes a singularity at Macusani, but leucogranite magma generation at low to intermediate pressures is known in the Variscan belt (Velay dome, Montel et al., 1986; Toledo and Peña Negra complexes, Barbero and Villaseca, 1992; Bea et al., 1999) as well as in the Himalayas (Visona et al., 2012). In the same way, temperatures in the Macusani source region, although elevated, are within the range considered possible for leucogranites (Montel et al., 1986; Scaillet et al., 1995). Therefore, the Macusani magmas were generated neither at exceptionally high temperatures nor at exceptionally low pressures. Peraluminous rhyolites equivalent to the Himalayan leucogranites occur in Tibet (Wang et al., 2012). In the Variscan belt, rhyolites analogous to rare-metal granites are known (e.g., the Richemont rhyolite, Raimbault and Burnol, 1998) so that a volcanic emplacement, although rare, is not exceptional for peraluminous silicic magmas. We note that the Central Andean Eastern Cordillera and the Himalayas share broad similarities in tectonic setting (continental collision), crustal structure (overthickened crust with intra-crustal low-velocity zones) and topography (high-altitude plateau). Both the Cordillera Oriental and Tibet are also characterized by widespread mafic K-UK magmatism broadly contemporaneous with peraluminous silicic rocks (Ding et al., 2003; Carlier et al., 2005). Lamprophyres are a systematic igneous component of the Variscan belt (Chalier and Sabourdy, 1987; Turpin et al., 1988; Soder and Romer, 2018), and deep crustal sections expose the genetic link between migmatites, granites and mafic, commonly K-UK, magma intrusions (Weisbrod et al., 1980; Bea et al., 1999, 2021; Castro et al., 2003). We conclude that the Macusani Volcanics provides a representative example of the generation of a strongly peraluminous silicic magma.
5.2 Crustal magma generation
The limitations and uncertainties of the intra-crustal model pointed out above, on the one hand, and the Macusani example, on the other hand, suggest that a mantle filiation is possible for felsic peraluminous magmas, as is the case for other silicic crustal magma types (see Sect. 2.3). Although we do not exclude the possibility of pure crustal melting (Fig. 1a), we note that most crustal magma types have a mixed, crust and mantle, origin and that the felsic peraluminous magmas do not make an exception.
A general crustal magma generation model incorporating the conclusion above is illustrated on Fig. 5. During late orogenic decompression and extension of a previously thickened crust such as in the Variscan belt, anatectic gneiss domes and migmatites develop. Intrusion of mafic mantle magmas (mostly of K-UK nature, i.e., vaugnerites, durbachites, sanukitoids) in the lower to middle crust provides additional heat and volatiles (Weisbrod et al., 1980; Bea et al., 1999, 2021; Castro et al., 2003; Moyen et al., 2017; Wolfram et al., 2019; Castro, 2020; Gomez-Frutos and Castro, 2022). Partial melting is triggered by crustal heat production assisted locally by the mafic mantle-derived magmas. This leads to the generation of strongly peraluminous crustal magmas ranging from felsic (leucogranites or MPG, e.g., Nabelek, 2020) to more mafic (cordierite monzogranites or CPG, e.g., Castro et al., 1999). At deeper levels, mafic (noritic and charnockitic) intrusions are generated (Vielzeuf et al., 2021). Hybridization between mantle magmas and crustal melts is minor and only local in migmatites and in MPG. It becomes more important in CPG, which typically host dark microgranular enclaves (Fig. 5). The mafic noritic and charnockitic lower crustal intrusions are products of high degrees of hybridization between mantle and crustal melts (Vielzeuf et al., 2021). KCG originate from differentiation of potassic and magnesian mafic magmas (vaugnerites) combined with melting/assimilation of crustal rocks (Moyen et al., 2017; Castro, 2020). Thus, most crustal granite magmas can be viewed as hybrid products. Variations in the proportion and nature of the crust and mantle end-members are the main factors at the origin of the magma compositional diversity. The model of Fig. 5 may be seen as an intermediate between the two in Fig. 1. It contains elements (e.g., migmatites) that are typical of the intra-crustal melting model and others (e.g., mafic mantle-derived magmas) that are typical of the basaltic origin model. However, the influence of mafic magmas is local in Fig. 5 rather than general as in Fig. 1b.
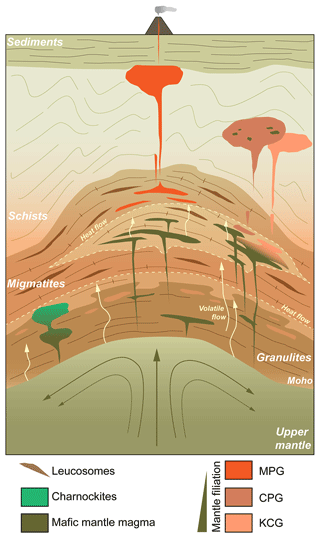
Figure 5General model for silicic magma generation during late orogenic decompression and extension of a previously thickened crust. Upwelling of the mantle increases conductive heat transfer to the lower crust. Heat is also advected from mafic mantle magmas, mostly of K-UK nature, emplaced at mid-crustal levels. Both the mantle and mafic magmas provide volatiles so that dehydration-melting and fluid-present melting are combined. Heat and volatile supply promote episodes of fast local crustal melt production in both migmatites and granulites. In migmatites, strongly peraluminous melts are generated at relatively low temperatures. The crustal source component is largely dominant; hybridization between mantle magmas and anatectic melts is only local. Leucogranitic melts (MPG) crystallize in situ (leucosomes), whereas others form magma batches that contribute to the growth of shallow granitic plutons, being more rarely erupted. Cordierite monzogranites and granodiorites (CPG) bear the mark of a stronger mantle filiation (mafic microgranular enclaves); they are generated at high temperatures by melting and reactive assimilation of crustal rocks by the mantle magmas (Castro et al., 1999). Potassic calcalkaline granites (KCG) result from differentiation of potassic and magnesian mafic magmas (vaugnerites) combined with melting/assimilation of crustal rocks (Moyen et al., 2017; Castro, 2020). In granulites, noritic and charnockitic intrusions represent high-temperature magmas formed by high degrees of hybridization between mantle-derived and lower crustal melts (Vielzeuf et al., 2021). So, most crustal granite magmas are hybrid products. See also text.
Further work is necessary to test the applicability of this model to the Himalayan leucogranites, but we note that concepts for their origin are rapidly evolving. Wu et al. (2020) emphasized crystal fractionation as an important mechanism controlling the geochemistry of Himalayan leucogranites, instead of partial melting (see also Scaillet et al., 1990). Melt was inferred to be generated at relatively low pressures following assimilation of metapelitic country rocks rather than by muscovite DM during decompression of overthickened crust as commonly proposed (Nabelek, 2020). Primary Himalayan magmas were considered metaluminous or only slightly peraluminous rather than as strongly peraluminous, with the derivation of leucogranites from more mafic parental magmas being supported by the occurrence of dioritic enclaves in some plutons (Zheng et al., 2016). The model of Wu et al. (2020) differs in key points from the intra-crustal melting (e.g., Nabelek, 2020) but shares several aspects with Macusani (melt production mechanisms, importance given to magma fractionation, involvement of mafic magmas, Fig. 5), and it can be viewed as representative of new ideas in the generation of leucogranites.
Some aspects illustrated in Fig. 5 are worth being emphasized, in particular the thermal and temporal aspects. Peraluminous silicic magmas may represent responses of crustal metasedimentary protoliths to excursions (both in temperature and volatile supply) driven by local intrusions of mafic mantle magmas. Detection of those very short processes, instantaneous at the scale of long-lived migmatites and granitic plutons, represents a challenge (Vielzeuf et al., 2021). The hypothesis is that peraluminous magma generation is associated with multiple but short transient partial melting events in which melting kinetics exerts an important control. This would help to explain several puzzling features such as the mineralogical and geochemical homogeneity of leucogranites compared to migmatites as noted in the Himalayan case.
5.3 Silicic magma generation
Hildreth (1981) presented a fundamentally basaltic view of lithospheric magmatism with Himalayan two-mica leucogranites representing the exception proving the basaltic rule. One fundamental result of the present contribution is that a mantle filiation is recognized for peraluminous leucogranitic magmas as for other crustal magma types. Therefore, singularity can be dropped, and this opens the perspective of unification of concepts for silicic magma genesis. The basaltic origin model accounts for the larger part of granite and crust production. It explicitly attributes a role to the mantle and crust (and to their mutual interactions) in silicic magma genesis (Fig. 1b). In comparison, the intra-crustal melting model basically dismisses the mantle contribution to magma generation and, so, appears less general (Fig. 1a). Therefore, the basaltic origin holds a greater promise than the intra-crustal model. It is flexible, can be adapted to examples of magma generation where the crustal component is dominant (Fig. 5) and could well form the basis of a general model for granite generation, applicable to most magma types. Unification of silicic magma generation models in the frame of the basaltic origin model appears within reach. This would represent an important step forward in the maturation of scientific ideas on this classical geological question.
The main conclusions of this paper are the following.
-
Source regions of silicic magmas are spatially heterogeneous at various scales, and they have long lifetimes (> 1–10 Ma or more). They must be considered open to external components; mafic intrusions; and/or fluids, heat and mass supply being intimately linked. A fundamental evolution towards a dynamic and open-system source rock concept is encouraged.
-
There are limitations and shortcomings in the present-day version of the intra-crustal melting model. Several key assumptions need careful reevaluation. These criticisms emphasize the need for future research efforts on partial melting and the origin of crustal granites.
-
The Macusani case demonstrates that, as for other crustal magma types, a mantle filiation is possible for peraluminous leucogranitic magmas. Therefore, these do not represent an exception among crustal magma types. Although the possibility of pure crustal melting is not excluded, a mixed, crust and mantle, origin is recognized for most crustal magma types.
-
The basaltic origin model, which accounts for the larger part of granite and crust production, forms the basis of a general model for granite generation, which is applicable to most magma types. Unification of silicic magma generation models appears within reach.
Data in Tables 1 and 2 are original data obtained by the authors for the present study. The other data used in Figs. 3 and 4 are from the literature and can be made available upon request.
MP wrote the text and prepared the figures and tables with contributions from JASM. AV performed the experiments and analyses. BS reviewed, corrected and improved the text.
The contact author has declared that none of the authors has any competing interests.
Publisher’s note: Copernicus Publications remains neutral with regard to jurisdictional claims made in the text, published maps, institutional affiliations, or any other geographical representation in this paper. While Copernicus Publications makes every effort to include appropriate place names, the final responsibility lies with the authors.
This article is part of the special issue “Probing the Earth: magma and fluids, a tribute to the career of Michel Pichavant”. It is a result of the Magma & Fluids workshop, Orléans, France, 4–6 July 2022.
This paper summarizes a scientific evolution that has matured for years and has benefited from contributions of many teachers, colleagues and students including Francis Albarède, Pierre Barbey, William Brown, Wayne Burnham, Antonio Castro, Bernard Charoy, Michel Cuney, Roberto Dall'Agnol, Donald Dingwell, Eric Gloaguen, Tahar Hammouda, François Holtz, Daniel Kontak, Nicole Le Breton, Patrick Le Fort, David Manning, Christian Marignac, Jean-Marc Montel, Bjorn Mysen, Rolf Romer, Daniel Vielzeuf and Alain Weisbrod. George Bergantz is acknowledged for sharing his views about basaltic underplating. Discussions with Olivier Bachmann, Jannick Ingrin and Jean-François Moyen stimulated the writing. Fabrice Gaillard, the organizers, speakers and all the participants of the Orléans workshop in July 2022 are gratefully acknowledged.
Recent work on the origin of granites at Orléans has been supported by the ANR VARPEG project, the LABEX VOLTAIRE project and the EQUIPEX PLANEX project and at Hanover by the SPP 2238 DOME grant no. 1337/49-1.
This paper was edited by Francois Holtz and reviewed by Eric Christiansen and Roberto Weinberg.
Albarède, F., Dupuis, C., and Taylor Jr., H. P.: evidence for non-cogenetic magmas associated in a 300 Ma old concentric pluton at Ploumanac'h (Brittany, France), J. Geol. Soc. Lond., 137, 641–647, 1980.
Annen, C., Blundy, J. D., and Sparks, R. S. J.: The genesis of intermediate and silicic magmas in deep crustal hot zones, J. Petrol., 47, 505–539, 2006.
Annen, C., Blundy, J. D., Leuthold, J., and Sparks, R. S. J.: Construction and evolution of igneous bodies: towards an integrated perspective of crustal magmatism, Lithos, 230, 206–221, 2015.
Aranovich, L. Y., Newton, R. C., and Manning, C. E.: Brine-assisted anatexis: experimental melting in the system haplogranite–H2O–NaCl–KCl at deep-crustal conditions, Earth Planet. Sc. Lett., 374, 111–120, 2013.
Aranovich, L. Y., Makhluf, A. R., Manning, C. E., and Newton, R. C.: Dehydration melting and the relationship between granites and granulites, Precambrian Res., 253, 26–37, 2014.
Bacon, C. R.: Magmatic inclusions in silicic and intermediate volcanic rocks, J. Geophys. Res., 91, 6091–6112, 1986.
Barbarin, B.: A review of the relationships between granitoid types, their origins and their geodynamic environments, Lithos, 46, 605–626, 1999.
Barbarin, B. and Didier, J.: Genesis and evolution of mafic magmatic enclaves through various types of interactions between coeval felsic and mafic magmas, T. Roy. Soc. Edin.-Earth, 83, 145–153, 1992.
Barbero, L. and Villaseca, C.: The Layos granite, hercynian complex of Toledo (Spain): an example of parautochthonous restite-rich granite in a granulitic area, T. Roy. Soc. Edin.-Earth, 83, 127–138, 1992.
Barbey, P., Allé, P., Brouand, M., and Albarède, F.: Rare earth element patterns in zircons from the Manaslu granite and Tibetan Slab migmatites (Himalaya): insights in the origin and evolution of crustally-derived magmas, Chem. Geol., 125, 1–17, 1995.
Barbey, P., Brouand, M., Le Fort, P., and Pêcher, A.: Granite-migmatite genetic link: the example of the Manaslu granite and Tibetan Slab migmatites in central Nepal, Lithos, 38, 63–79, 1996.
Barbey, P., Nachit, H., and Pons, J.: Magma–host interactions during differentiation and emplacement of a shallow-level, zoned granitic pluton (Tarcouate pluton, Morocco): implications for magma emplacement, Lithos, 58, 125–143, 2001.
Bartoli, O. and Carvalho, B. B.: Anatectic granites in their source region: A comparison between experiments, thermodynamic modelling and nanogranitoids, Lithos, 402–403, 106046, https://doi.org/10.1016/j.lithos.2021.106046, 2021.
Bartoli, O., Acosta-Vigil, A., Cesare, B., Remusat, L., Gonzalez-Cano, A., Wälle, M., Tajčmanová, L., and Langone, A.: Geochemistry of Eocene-Early Oligocene low-temperature crustal melts from Greater Himalayan Sequence (Nepal): a nanogranitoid perspective, Contrib. Mineral. Petr., 174, 82, https://doi.org/10.1007/s00410-019-1622-2, 2019.
Bea, F.: The sources of energy for crustal melting and the geochemistry of heat producing elements, Lithos, 153, 278–291, 2012.
Bea, F., Montero, P., and Molina, J. F.: Mafic precursors, peraluminous granitoids, and late lamprophyres in the Avila batholith: a model for the generation of Variscan batholiths in Iberia, J. Geol., 107, 399–419, 1999.
Bea, F., Gallastegui, G., Montero, P., Molina, J.-F., Scarrow, J., Cuesta, A., and Gonzalez-Menedez, L.: Contrasting high-Mg, high-K rocks in Central Iberia: the appinite–vaugnerite conundrum and their (non-existent) relation with arc magmatism, J. Iberian Geol., 47, 235–261, 2021.
Bergantz, G. W. and Dawes, R.: Aspects of magma generation and ascent in continental lithosphere, in: Magmatic Systems, edited by: Ryan, M. P., Academic Press, 291–317, https://doi.org/10.1016/s0074-6142(09)60101-7, 1994.
Best, M. G., Christiansen, E. H., de Silva, S., and Lipman, P. W.: Slab-rollback ignimbrite flareups in the southern Great Basin and other Cenozoic American arcs: A distinct style of arc volcanism, Geosphere, 12, 1095–1135, 2016.
Bonin, B.: Do coeval mafic and felsic magmas in post-collisional to within-plate regimes necessarily imply two contrasting, mantle and crustal, sources? A review, Lithos, 78, 1–24, 2004.
Bowen, N. L.: The behavior of inclusions in igneous magma, J. Geol., XXX, 513–570, 1922.
Brown, M.: Retrograde processes in migmatites and granulites revisited, J. Metamorph. Geol., 20, 25–40, 2002.
Brown, M.: Granites: from genesis to emplacement, Geol. Soc. Am. Bull., 125, 1079–113, 2013.
Burgess, S. D. and Miller, J. S.: Construction, solidification and internal differentiation of a large felsic arc pluton: Cathedral Peak granodiorite, Sierra Nevada Batholith, in: Dynamics of Crustal Magma Transfer, Storage and Differentiation, edited by: Annen, C. and Zellmer, G. F., Geological Society, London, Special Publications, Vol. 304, 203–233, https://doi.org/10.1144/sp304.11, 2008.
Cadoux, A., Scaillet, B., Druitt, T. H., and Deloule, E.: Magma storage conditions of large Plinian eruptions of Santorini volcano (Greece), J. Petrol., 55, 1129–1171, 2014.
Carlier, G., Lorand, J.-P., Liégeois, J.-P., Fornari, M., Soler, P., Carlotto, V., and Cardenas, J.: Potassic-ultrapotassic mafic rocks delineate two lithospheric mantle blocks beneath the southern Peruvian Altiplano, Geology, 33, 601–604, 2005.
Carvalho, B. B., Sawyer, E. S., and Janasi, V. A.: Enhancing maficity of granitic magma during anatexis: Entrainment of infertile mafic lithologies, J. Petrol., 58, 1333–1362, 2017.
Carvalho, B. B., Bartoli, O., Ferri, F., Cesare, B., Ferrero, S., Remusat, L., Capizzi, L. S., and Poli, S.: Anatexis and fluid regime of the deep continental crust: new clues from melt and fluid inclusions in metapelitic migmatites from Ivrea Zone (NW Italy), J. Metamorph. Geol., 37, 951–975, 2019.
Castro, A.: The dual origin of I-type granites: the contribution from experiments, in: Post-Archean Granitic Rocks: Contrasting Petrogenetic Processes and Tectonic Environments, edited by: Janousek, V., Bonin, B., Collins, W. J., Farina, F., and Bowden, P., Geological Society, London, Special Publications, Vol. 491, 101–145, https://doi.org/10.1144/sp491-2018-110, 2020.
Castro, A., Patiño Douce, A. E., Corretge, L. G., de la Rosa, J. D., El-Biad, M., and El-Hmidi, H.: Origin of peraluminous granites and granodiorites, Iberian massif, Spain: an experimental test of granite petrogenesis, Contrib. Mineral. Petr., 135, 255–276, 1999.
Castro, A., Corretge, L. G., de la Rosa, J. D., Fernandez, C., Lopez, S., Garcia-Moreno, O., and Chacon, H.: The appinite-migmatite complex of Sanabria, NW Iberian Massif, Spain, J. Petrol., 44, 1309–1344, 2003.
Chalier, M. and Sabourdy, G.: Les lamprophyres du granite hyperalumineux de Saint-Sylvestre (Limousin, Massif Central français). Caractères pétrologiques et origine, C.R. Acad. Sci. Paris, 305, 99–105, 1987.
Chappell, B. W. and Stephens, W. E.: Origin of infracrustal (I-type) granite magmas, T. Roy. Soc. Edin.-Earth, 79, 71–86, 1988.
Chappell, B. W. and White, A. J. R.: Two contrasting granite types, Pacific Geology, 8, 173–174, 1974.
Chappell, B. W., White, A. J. R., and Wyborn, D.: The importance of residual source material (restite) in granite petrogenesis, J. Petrol., 28, 1111–1138, 1987.
Cheilletz, A., Clark, A. H., Farrar, E., Arroyo Pauca, G., Pichavant, M., and Sandeman, H. A.: Volcano-stratigraphy and 40Ar/39Ar geochronology of the Macusani ignimbrite field: monitor of the Miocene geodynamic evolution of the Andes of southeast Peru, Tectonophys., 205, 307-327, 1992.
Christiansen, E. H. and McCurry, M.: Contrasting origins of Cenozoic silicic volcanic rocks from the western Cordillera of the United States, Contrib. Mineral. Petr., 70, 251–267, 2008.
Christiansen, E. H., Haapala, I., and Hart, G. L.: Are Cenozoic topaz rhyolites the erupted equivalents of Proterozoic rapakivi granites? Examples from the western United States and Finland, Lithos, 97, 219–246, 2007.
Clark, C., Fitzsimons, I. C. W., Healy, D., and Harley, S. L.: How does the continental crust get really hot?, Elements, 7, 235–240, 2011.
Clarke, D. B.: The mineralogy of peraluminous granites: A review, Can. Mineral., 19, 3–17, 1981.
Clarke, D. B.: The origins of strongly peraluminous granitoid rocks, Can. Mineral., 57, 529–550, 2019.
Clemens, J. C. and Vielzeuf, D.: Constraints on melting and magma production in the crust, Earth Planet. Sc. Lett., 86, 287–306, 1987.
Clemens, J. C. and Wall, V. J.: Origin and evolution of a peraluminous silicic ignimbrite suite: the Violet Town Volcanics, Contrib. Mineral. Petr., 88, 354–371, 1984.
Collins, W. J.: Lachlan Fold Belt granitoids: products of three-component mixing, T. Roy. Soc. Edin.-Earth, 87, 171–181, 1996.
Collins, W. J., Beams, S. D., White, A. J. R., and Chappell, B. W.: Nature and origin of A-type granites with particular reference to southeastern Australia, Contrib. Mineral. Petr., 80, 189–200, 1982.
Connolly, J. A. D. and Cesare, B.: C-O-H-S fluid composition and oxygen fugacity in graphitic metapelites, J. Metamorph. Geol., 11, 379–388, 1993.
Conrad, W. K., Nicholls, I. A., and Wall, V. J.: Water-saturated and -undersaturated melting of metaluminous and peraluminous crustal compositions at 10 kb: Evidence for the origin of silicic magmas in the Taupo Volcanic Zone, New Zealand, and other occurrences, J. Petrol., 29, 765–803, 1988.
Couzinié, S., Laurent, O., Moyen, J.-F., Zeh, A., Bouilhol, P., and Villaros, A.: Post-collisional magmatism: crustal growth not identified by zircon Hf–O isotopes, Earth Planet. Sc. Lett., 456, 182–195, 2016.
Creaser, R. A., Price, R. C., and Wormald, R. J.: A-type granites revisited: Assessment of a residual-source model, Geology, 19, 163–166, 1991.
Cuney, M. and Barbey, P.: Uranium, rare metals, and granulite-facies metamorphism, Geosci. Front., 5, 729–745, 2014.
Dall'Agnol, R., Scaillet, B., and Pichavant, M.: An experimental study of a lower Proterozoic A-type granite from the eastern Amazonian craton, Brazil, J. Petrol., 40, 1673–1698, 1999.
Dasgupta, R., Jackson, M. G., and Lee, C.-T. A.: Major element chemistry of ocean island basalts – conditions of mantle melting and heterogeneity of mantle source, Earth Planet. Sc. Lett., 289, 377–392, 2010.
Ding, L., Kapp, P., Zhong, D., and Deng, W.: Cenozoic volcanism in Tibet: evidence for a transition from oceanic to continental subduction, J. Petrol., 44, 1833–1865, 2003.
Dorais, M. J. and Campbell, S.: Peritectic and phenocrystic garnet accumulation and the origin of strongly peraluminous granitic rocks: The Flagstaff Lake Igneous Complex, Maine, Lithos, 418–419, 106680, https://doi.org/10.1016/j.lithos.2022.106680, 2022.
Dufek, J. and Bergantz, G. W.: Lower crustal magma genesis and preservation: a stochastic framework for the evaluation of basalt–crust interaction, J. Petrol., 46, 2167–2195, 2005.
Eggler, D. H.: Principles of melting of hydrous phases in silicate melt, Carnegie I. Wash., 72, 491–495, 1973.
Eichelberger, J. C.: Andesites in island arcs and continental margins: relationship to crustal evoIution, B. Volcanol., 41, 480–500, 1978.
Eichelberger, J. C., Chertkoff, D. G., Dreher, S. T., and Nye, C. J.: Magmas in collision: rethinking chemical zonation in silicic magmas, Geology, 28, 603–606, 2000.
Eichelberger, J. C., Izbekov, P. E., and Browne, B. L.: Bulk chemical trends at arc volcanoes are not liquid lines of descent, Lithos, 87, 135–154, 2006.
England, P. E. and Thompson, A.: Some thermal and tectonic models for crustal melting in continental collision zones, in: Collision Tectonics, edited by: Coward, M. P. and Ries, A. C., Geological Society Special Publication, Vol. 19, 83–94, https://doi.org/10.1144/gsl.sp.1986.019.01.05, 1986.
Erdmann, S., Jamieson, R. A., and Macdonald, M. A.: Evaluating the origin of garnet, cordierite, and biotite in granitic rocks: a case study from the South Mountain Batholith, Nova Scotia, J. Petrol., 50, 1477–1503, 2009.
Farina, F., Dini, A., Rocchi, S., and Stevens, G.: Extreme mineral-scale Sr isotope heterogeneity in granites by disequilibrium melting of the crust, Earth Planet. Sc. Lett., 399, 103–115, 2014.
Fowler, M. B., Henney, P. J., Darbyshire, D. P. F., and Greenwood, P. B.: Petrogenesis of high Ba-Sr granites: the Rogart pluton, Sutherland, J. Geol. Soc. Lond., 158, 521–534, 2001.
France-Lanord, C., Sheppard, S. M. F., and Le Fort, P.: Hydrogen and oxygen isotope variations in the High Himalaya peraluminous Manaslu leucogranite: evidence for heterogeneous sedimentary source, Geochim. Cosmochim. Ac., 52, 513–526, 1988.
Gao, L.-E., Zeng, L., and Asimow, P. D.: Contrasting geochemical signatures of fluid-absent versus fluid-fluxed melting of muscovite in metasedimentary sources: the Himalayan leucogranites, Geology, 45, 39–42, 2017.
García-Arias, M.: Decoupled Ca and Fe + Mg content of S-type granites: An investigation on the factors that control the Ca budget of S-type granites, Lithos, 318–319, 30–46, 2018.
García-Arias, M. and Stevens, G.: Phase equilibrium modelling of granite magma petrogenesis: an evaluation of the magma compositions produced by crystal entrainment in the source, Lithos, 277, 131–153, 2017.
Gardien, V., Thompson, A. B., Grujic, D., and Ulmer, P.: Experimental melting of biotite + plagioclase + quartz + muscovite assemblages and implications for crustal melting, J. Geophys. Res., 100, 15581–15591, 1995.
Gebelin, A., Roger, F., and Brunel, M.: Syntectonic crustal melting and high-grade metamorphism in a transpressional regime, Variscan Massif Central, France, Tectonophysics, 477, 229–243, 2009.
Gill, J. B.: Orogenic andesites and plate tectonics, Springer, 390 pp., https://doi.org/10.1007/978-3-642-68012-0, 1981.
Gomez-Frutos, D. and Castro, A.: Sanukitoid crystallization relations at 1.0 and 0.3 GPa, Lithos, 414–415, 106632, https://doi.org/10.1016/j.lithos.2022.106632, 2022.
Gomez-Frutos, D., Castro, A., and Gutierrez-Alonso, G.: Post-collisional batholiths do contribute to continental growth, Earth Planet. Sc. Lett., 603, 117978, https://doi.org/10.1016/j.epsl.2022.117978, 2023.
Gray, W., Glazner, A., Coleman, D. S., and Bartley, J. M.: Long-term geochemical variability of the Late Cretaceous Tuolumne Intrusive Suite, central Sierra Nevada, California, in: Dynamics of Crustal Magma Transfer, Storage and Differentiation, edited by: Annen, C. and Zellmer, G. F., Geological Society, London, Special Publications, Vol. 304, 183–201, https://doi.org/10.1144/sp304.10, 2008.
Guillot, S. and Le Fort, P.: Geochemical constraints on the bimodal origin of High Himalayan leucogranites, Lithos, 35, 221–234, 1995.
Hammouda, T., Pichavant, M., and Chaussidon, M.: Isotopic equilibration during partial melting: an experimental test of the behaviour of Sr, Earth Planet. Sc. Lett., 144, 109–121, 1996.
Harris, N. B. W. and Inger, S.: Trace element modelling of pelite-derived granites, Contrib. Mineral. Petr., 110, 46–56, 1992.
Harris, N., Vance, D., and Ayres, M.: From sediment to granite: timescales of anatexis in the upper crust, Chem. Geol., 162, 155–167, 2000.
Hildreth, W.: Gradients in silicic magma chambers. Implications for lithospheric magmatism, J. Geophys. Res., 86, 10153–10192, 1981.
Hildreth, W. and Moorbath, S.: Crustal contribution to arc magmatism in the Andes of Central Chile, Contrib. Mineral. Petr., 98, 455–489, 1988.
Hogan, J. P. and Sinha, A. K.: The effect of accessory minerals on the redistribution of lead isotopes during crustal anatexis: a model, Geochim. Cosmochim. Ac., 55, 335–348, 1991.
Holland, T. J. B. and Powell, R.: An improved and extended internally consistent thermodynamic dataset for phases of petrological interest, involving new equations of state for solids, J. Metamorph. Geol., 29, 333–383, 2011.
Holloway, J. R.: Fluids in the evolution of granitic magmas: Consequences of finite CO2 solubility, Geol. Soc. Am. Bull., 87, 1513–1518, 1976.
Inger, S. and Harris, N.: Geochemical constraints on leucogranite magmatism in the Langtang Valley, Nepal Himalaya, J. Petrol., 34, 345–368, 1993.
Janoušek, V., Hanžl, P., Svojtka, M., Hora, J. M., Erban Kochergina, Y. V., Gadas, P., Holub, F. V., Gerdes, A., Verner, K., Hrdličková, K., Daly, J. S., and Buriánek, D.: Ultrapotassic magmatism in the heyday of the Variscan Orogeny: the story of the Třebíč Pluton, the largest durbachitic body in the Bohemian Massif, Int. J. Earth Sci., 109, 1767–1810, 2020.
Jaupart, C. and Provost, A.: Heat focussing, granite genesis and inverted metamorphic gradients in continental collision zones, Earth Planet. Sc. Lett., 73, 385–397, 1985.
Johannes, W. and Holtz, F.: Petrogenesis and Experimental Petrology of Granitic Rocks, Springer-Verlag, 301 pp., https://doi.org/10.1007/978-3-642-61049-3, 1996.
Juteau, M., Michard, A., and Albarède, F.: The Pb-Sr-Nd isotope geochemistry of some recent circum-Mediterranean granites, Contrib. Mineral. Petr., 92, 331–340, 1986.
Le Fort, P.: Himalaya: the collided range. Present knowledge of the continental arc, Am. J. Sci., 275A, 1–44, 1975.
Le Fort, P.: Manaslu leucogranite: a collision signature of the Himalaya. A model for its genesis and emplacement, J. Geophys. Res., 86, 10545–10568, 1981.
Le Fort, P., Cuney, M., Deniel, C., France-Lanord, C., Sheppard, S. M. F., Upreti, B. N., and Vidal, P.: Crustal generation of the Himalayan leucogranites, Tectonophysics, 134, 39–57, 1987.
Linnen, R. L. and Cuney, M.: Granite-related rare-element deposits and experimental constraints on Ta-Nb-W-Sn-Zr-Hf mineralization, in Rare-element Geochemistry and Mineral Deposits, edited by: Linnen, R. L. and Samson, I. M., Geological Association of Canada, Ottawa, 45–68, 2005.
London, D.: Reply to Thomas and Davidson on “A petrologic assessment of internal zonation in granitic pegmatites” (London, 2014a), Lithos, 212–215, 469–484, 2015.
Ma, Y. and Clayton, R. W.: The crust and uppermost mantle structure of Southern Peru from ambient noise and earthquake surface wave analysis, Earth Planet. Sc. Lett., 395, 61–70, 2014.
Manning, C. E. and Aranovich, L. Y.: Brines at high pressure and temperature: thermodynamic, petrologic and geochemical effects, Precambrian Res., 253, 6–16, 2014.
Marxer, F., Ulmer, P., and Müntener, O.: Polybaric fractional crystallisation of arc magmas: an experimental study simulating trans-crustal magmatic systems, Contrib. Mineral. Petr., 177, 3, https://doi.org/10.1007/s00410-021-01856-8, 2022.
Mehnert, K. R.: Migmatites and the origin of granitic rocks, Elsevier, 393 pp., 1968.
Michaud, J. A.-S. and Pichavant, M.: Magmatic fractionation and the magmatic-hydrothermal transition in rare metal granites: evidence from Argemela (Central Portugal), Geochim. Cosmochim. Ac., 289, 130–157, 2020.
Michaud, J. A.-S., Pichavant, M., and Villaros, A.: Rare elements enrichment in crustal peraluminous magmas: insights from partial melting experiments, Contrib. Mineral. Petr., 176, 96, https://doi.org/10.1007/s00410-021-01855-9, 2021.
Montel, J.-M., Weber, C., Barbey, P., and Pichavant, M.: Thermo-barométrie du domaine anatectique du Velay (Massif Central, France) et conditions de genèse des granites tardi-migmatitiques, C.R. Acad. Sci. Paris, 302, 647–652, 1986.
Montel, J.-M., Didier, J., and Pichavant, M.: Origin of surmicaceous enclaves in intrusive granites, in: Enclaves and Granite Petrology, edited by: Didier, J. and Barbarin, B., Elsevier, 509–528, 1991.
Moyen, J.-F., Laurent, O., Chelle-Michou, C., Couzinié, S., Vanderhaeghe, O., Zeh, A., Villaros, A., and Gardien, V.: Collision vs. subduction-related magmatism: two contrasting ways of granite formation and implications for crustal growth, Lithos, 277, 154–177, 2017.
Moyen, J.-F.: Granites and crustal heat budget, in: Post-Archean Granitic Rocks: Contrasting Petrogenetic Processes and Tectonic Environments, edited by: Janousek, V., Bonin, B., Collins, W. J., Farina, F., and Bowden, P., Geological Society, London, Special Publications, Vol. 491, 77–100, 2020.
Moyen, J.-F., Janousek, V., Laurent, O., Bachmann, O, Jacob, J.-B., Farina, F., Fiannacca, P, and Villaros, A.: Crustal melting vs. fractionation of basaltic magmas: Part 1, granites and paradigms, Lithos, 402–403, 106291, https://doi.org/10.1016/j.lithos.2021.106291, 2021.
Nabelek, P. I.: Petrogenesis of leucogranites in collisional orogens, in: Post-Archean Granitic Rocks: Contrasting Petrogenetic Processes and Tectonic Environments, edited by: Janousek, V., Bonin, B., Collins, W. J., Farina, F., and Bowden, P., Geological Society, London, Special Publications, Vol. 491, 179–207, https://doi.org/10.1144/sp491-2018-181, 2020.
Nabelek, P. I. and Nabelek, J. L.: Thermal characteristics of the Main Himalaya Thrust and the Indian lower crust with implications for crustal rheology and partial melting in the Himalaya orogen, Earth Planet. Sc. Lett., 395, 116–123, 2014.
Nabelek, P. I., Russ-Nabelek, C., and Haeussler, G. T.: Stable isotope evidence for the petrogenesis and fluid evolution in the Proterozoic Harney Peak leucogranite, Black Hills, South Dakota, Geochim. Cosmochim. Ac., 56, 403–417, 1992.
Newton, R. C.: Young and old granulites: a volatile connection, J. Geol., 128, 395–413, 2020.
Nicoli, G., Stevens, G., Moyen, J.-F., Vézinet, A., and Mayne, M.: Insights into the complexity of crustal differentiation: K2O-poor leucosomes within metasedimentary migmatites from the Southern Marginal Zone of the Limpopo Belt, South Africa, J. Metamorph. Geol., 35, 999–1022, 2017.
Noble, D. C., Vogel, T. A., Peterson, P. S., Landis, G. P., Grant, N. K., Jezek, P. A., and McKee, E. H.: Rare-element-enriched, S-type ash-flow tuffs containing phenocrysts of muscovite, andalusite, and sillimanite, southeastern Peru, Geology, 12, 35–39, 1984.
Patiño-Douce, A. E.: Experimental generation of hybrid silicic melts by reaction of high-Al basalt with metamorphic rocks, J. Geophys. Res., 100, 15623–15639, 1995.
Patiño-Douce, A. E. and Harris, N.: Experimental constraints on Himalayan anatexis, J. Petrol., 39, 689–710, 1998.
Pichavant, M.: Experimental crystallization of the Beauvoir granite as a model for the evolution of Variscan rare metal magmas, J. Petrol., 63, 1–28, 2022.
Pichavant, M. and Macdonald, R.: Crystallization of primitive basaltic magmas at crustal pressures and genesis of the calc-alkaline igneous suite: experimental evidence from St Vincent, Lesser Antilles arc, Contrib. Mineral. Petr., 154, 535–558, 2007.
Pichavant, M., Valencia Herrera, J., Boulmier, S., Briqueu, L., Joron, J.-L., Juteau, M., Michard, A., Sheppard, S. M. F., Treuil, M., and Vernet, M.: The Macusani glasses, SE Peru: evidence of chemical fractionation in peraluminous magmas, in: Magmatic Processes: Physicochemical Principles, edited by: Mysen, B. O., The Geochemical Society, University Park, 359–373, 1987.
Pichavant, M., Kontak, D., J., Valencia Herrera, J., and Clark, A. H.: The Miocene-Pliocene Macusani Volcanics, I. Mineralogy and magmatic evolution of a two-mica aluminosilicate-bearing ignimbrite suite, Contrib. Mineral. Petr., 100, 300–324, 1988a.
Pichavant, M., Kontak, D. J., Briqueu, L., Valencia Herrera, J., and Clark, A. H.: The Miocene-Pliocene Macusani Volcanics, II. Geochemistry and origin of a felsic peraluminous magma, Contrib. Mineral. Petr., 100, 325–338, 1988b.
Pichavant, M., Di Carlo, I., Le Gac, Y., Rotolo, S. G., and Scaillet, B.: Experimental Constraints on the Deep Magma Feeding System at Stromboli Volcano, Italy, J. Petrol., 50, 601–624, 2009.
Pichavant, M., Weber, C., and Villaros, A.: Effect of anorthite on granite phase relations: experimental data and models, C.R. Geosci., 351, 540–550, 2019.
Pichavant, M., Erdmann, S., Kontak, D. J., Michaud, J. A.-S., and Villaros, A.: Trace element partitioning in strongly peraluminous rare-metal silicic magmas – Implications for fractionation processes and for the origin of the Macusani Volcanics (SE Peru), Geochim. Cosmochim. Ac., 365, 229–252, https://doi.org/10.1016/j.gca.2023.11.021, 2023.
Pin, C. and Vielzeuf, D.: Granulites and related rocks in Variscan median Europe: a dualistic interpretation, Tectonophys., 93, 47–74, 1983.
Pinet, C. and Jaupart, C.: A thermal model for the distribution in space and time of the Himalayan granites, Earth Planet. Sc. Lett., 84, 87–99, 1987.
Raimbault, L. and Burnol, L.: The Richemont rhyolite dyke, Massif Central, France: a subvolcanic equivalent of rare-metal granites, Can. Mineral., 36, 265–282, 1998.
Romer, R. L. and Pichavant, M.: Rare metal granites and pegmatites, in: Encyclopedia of Geology, 2nd Edn., edited by: Alderton, D. and Elias, S. A., Academic Press, 840–846, https://doi.org/10.1016/b978-0-08-102908-4.00003-5, 2021.
Sandeman, H. A. and Clark, A. H.: Glass-rich, cordierite-biotite rhyodacite, Valle Ninahuisa, Puno, SE Peru: petrological evidence for hybridization of “Lachlan S-type” and potassic mafic magmas, J. Petrol., 44, 355–385, 2003.
Sandeman, H. A. and Clark, A. H.: Commingling and mixing of S-type peraluminous, ultrapotassic and basaltic magmas in the Cayconi volcanic field, Cordillera de Carabaya, SE Peru, Lithos, 73, 187–213, 2004.
Scaillet, B. and Searle, M. P.: Mechanisms and timescales of felsic magma segregation, ascent and emplacement in the Himalaya, in: Channel Flow, Ductile Extrusion and Exhumation in Continental Collision Zones, edited by: Law, R. D., Searle, M. P., and Godin, L., Geological Society, London, Special Publications, 268, 293–308, https://doi.org/10.1144/gsl.sp.2006.268.01.14, 2006.
Scaillet, B., France-Lanord, C., and Le Fort, P.: Badrinath–Gangotri plutons (Garhwal, India): petrological and geochemical evidence for fractionation processes in High Himalayan leucogranite, J. Volcanol. Geoth. Res., 44, 163–188, 1990.
Scaillet, B., Pichavant, M., and Roux, J.: Experimental crystallization of leucogranite magmas, J. Petrol., 36, 663–705, 1995.
Scaillet, B., Holtz, F., and Pichavant, M.: Phase equilibrium constraints on the viscosity of silicic magmas. 1. Volcanic-plutonic comparison, J. Geophys. Res., 103, 27257–27266, 1998.
Sinigoi, S., Quick, J. E., Demarchi, G., and Klötzli, U. S.: Production of hybrid granitic magma at the advancing front of basaltic underplating: inferences from the Sesia Magmatic System (south-western Alps, Italy), Lithos, 252–253, 109–122, 2016.
Soder, C. L. and Romer, R. L.: Post-collisional potassic–ultrapotassic magmatism of the Variscan orogen: implications for mantle metasomatism during continental subduction, J. Petrol., 59, 1007–1034, 2018.
Stevens, G., Villaros, A., and Moyen, J.-F.: Selective peritectic garnet entrainment as the origin of geochemical diversity in S-type granites, Geology, 35, 9–12, 2007.
Tang, M., Wang, X.-L., Shu, X.-J., Wang, D., Yang, T., and Gopon, P.: Hafnium isotopic heterogeneity in zircons from granitic rocks: geochemical evaluation and modeling of “zircon effect” in crustal anatexis, Earth Planet. Sc. Lett., 389, 188–199, 2014.
Thompson, A.: Dehydration melting of pelitic rocks and the generation of H2O-undersaturated granitic liquids, Am. J. Sci., 282, 1567–1595, 1982.
Touret, J.: Le facies granulite en Norvège méridionale: II. Les inclusions fluides, Lithos, 4, 423–436, 1971.
Touret, J. L. R. and Huizenga, J. M.: Fluid-assisted granulite metamorphism: a continental journey, Gondwana Res., 21, 224–235, 2012.
Turpin, L., Velde, D., and Pinte, G.: Geochemical comparison between minettes and kersantites from the Western European Hercynian orogen: trace element and Pb-Sr-Nd isotope constraints on their origin, Earth Planet. Sc. Lett., 88, 73–86, 1988.
Turpin, L., Cuney, M., Friedrich, M., Bouchez, J.-L., and Aubertin, M.: Meta-igneous origin of Hercynian peraluminous granites in NW French Massif Central: implications for crustal history reconstructions, Contrib. Mineral. Petr., 104, 163–172, 1990.
Tuttle, O. F. and Bowen, N. L.: Origin of granite in the light of experimental studies in the system NaAlSi3O8-KAlSi3O8-SiO2-H2O, Geol. Soc. Am. Mem., 74, 153 pp., https://doi.org/10.1130/mem74-p1, 1958.
Vernon, R. H.: Problems in identifying restite in S-type granites of southeastern Australia, with speculations on sources of magma and enclaves, Can. Mineral., 45, 147–178, 2007.
Vielzeuf, D. and Vidal, P. (Eds.): Granulites and crustal evolution, Nato Science Series C, 396 pp., https://doi.org/10.1007/978-94-009-2055-2, 1990.
Vielzeuf, D., Paquette, J.-L., Clemens, J. C., Stevens, G., Gannoun, A., Suchorski, K., and Saul, A.: Age, duration and mineral markers of magma interactions in the deep crust: an example from the Pyrenees, Contrib. Mineral. Petr., 176, 39, https://doi.org/10.1007/s00410-021-01789-2, 2021.
Visona, D., Carosi, R., Montomoli, C., Tiepolo, M., and Peruzzo, L.: Miocene andalusite leucogranite in central-east Himalaya (Everest–Masang Kang area): Low-pressure melting during heating, Lithos, 144–145, 194–208, 2012.
Walker Jr., B. A., Bergantz, G. A., Otamendi, J. E., Ducea, M. N., and Cristofolini, E. A.: A MASH zone revealed: the mafic complex of the Sierra Valle Fertil, J. Petrol., 56, 1863–1896, 2015.
Wang, J.-M., Rubatto, D., and Zhang, J.-J.: Timing of partial melting and cooling across the Greater Himalayan crystalline complex (Nyalam, Central Himalaya): in-sequence thrusting and its implications, J. Petrol., 56, 1677–1702, 2015.
Wang, Q., Chung, S.-L., Li, X.-H., Wyman, D., Li, Z.-X., Sun, W.-D., Qiu, H.-N., Liu, Y.-S., and Zhu, Y.-T.: Crustal melting and flow beneath northern Tibet: evidence from mid-miocene to quaternary strongly peraluminous rhyolites in the Southern Kunlun Range, J. Petrol., 53, 2523–2566, 2012.
Weinberg, R. F. and Hasalova, P.: Water-fluxed melting of the continental crust: a review, Lithos, 212, 158–188, 2015.
Weisbrod, A., Pichavant, M., Marignac, C., Macaudière, J., and Leroy, J.: Relations structurales et chronologiques entre le magmatisme basique, les granitisations et l'évolution tectonométamorphique tardihercynienne dans les Cévennes Médianes, Massif Central Français, C.R. Acad. Sci., Série D, 291, 665–668, 1980.
White, A. J. R. and Chappell, B. W.: Ultrametamorphism and granitoid genesis, Tectonophys, 43, 7–22, 1977.
White, R. W., Powell, R., Holland, T. J. B., Johnson, T. E., and Green, E. C. R.: New mineral activity-composition relations for thermodynamic calculations in metapelitic systems, J. Metamorph. Geol., 32, 261–286, 2014.
Wolf, M. B., Romer, R. L., and Glodny, J.: Isotope disequilibrium during partial melting of metasedimentary rocks, Geochim. Cosmochim. Ac., 257, 163–183, 2019.
Wolfram, L. C., Weinberg, R. F., Hasalova, P., and Becchio, R.: How melt segregation affects granite chemistry: Migmatites from the Sierra de Quilmes, NW Argentina, J. Petrol., 58, 2339–2364, 2017.
Wolfram, L. C., Weinberg, R. F., Nebel, O., Hamza, K., Hasalova, P., Mikova, J., and Becchio, R.: A 60-Myr record of continental back-arc differentiation through cyclic melting, Nat. Geosci., 12, 215–219, 2019.
Wu, F.-Y., Liu, X.-C., Liu, Z.-C., Wang, R.-C., Xie, L., Wang, J.-M., Ji, W.-Q., Yang, L., Liu, C., Khanal, G. P., and He, S.-X.: Highly fractionated Himalayan leucogranites and associated rare-metal mineralization, Lithos, 352–353, 105319, https://doi.org/10.1016/j.lithos.2019.105319, 2020.
Yang, L., Liu, X.-C., Wang, J.-M., and Wua, F.-Y.: Is Himalayan leucogranite a product by in situ partial melting of the Greater Himalayan Crystalline? a comparative study of leucosome and leucogranite from Nyalam, southern Tibet, Lithos, 342–343, 542–556, 2019.
Yardley, B. W. D.: The role of water in the evolution of the continental crust, J. Geol. Soc. Lond., 166, 585–600, 2009.
Zeng, L., Asimow, P. D., and Saleeby, J. B.: Coupling of anatectic reactions and dissolution of accessory phases and the Sr and Nd isotope systematics of anatectic melts from a metasedimentary source, Geochim. Cosmochim. Ac., 69, 3671–3682, 2005.
Zheng, Y.-C., Hou, Z.-Q., Fu, A., Zhu, D.-C., Liang, W., and Xua, P.: Mantle inputs to Himalayan anatexis: insights from petrogenesis of the Miocene Langkazi leucogranite and its dioritic enclaves, Lithos, 264, 125–140, 2016.
- Abstract
- Introduction
- Granite generation models
- Critical review of the intra-crustal melting model
- A case example of peraluminous silicic magma generation: the Macusani Volcanics (SE Peru)
- Discussion
- Conclusions
- Data availability
- Author contributions
- Competing interests
- Disclaimer
- Special issue statement
- Acknowledgements
- Financial support
- Review statement
- References
- Abstract
- Introduction
- Granite generation models
- Critical review of the intra-crustal melting model
- A case example of peraluminous silicic magma generation: the Macusani Volcanics (SE Peru)
- Discussion
- Conclusions
- Data availability
- Author contributions
- Competing interests
- Disclaimer
- Special issue statement
- Acknowledgements
- Financial support
- Review statement
- References