the Creative Commons Attribution 4.0 License.
the Creative Commons Attribution 4.0 License.
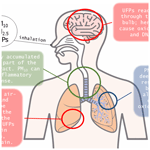
Mineralogy of the human brain: a review
Giulia Pia Servetto
Carissa Maria Root
Reto Gieré
Ruggero Vigliaturo
The human brain can both synthesize minerals in situ and accumulate exogenous phases from the surrounding environment. Some of the biogenic phases may represent evolutionary remains, whereas others are crucial for many physiological functions of the neurological system. Yet an excess concentration of these minerals in the brain may be a precursor and/or a consequence of several neurodegenerative conditions, such as Alzheimer's and Parkinson's diseases. In this regard, there is very little in the literature that is focused on the minerals and mineraloids present in the human brain and on their physicochemical state. The gap in the literature is particularly wide for nanoscale and sub-nanoscale compounds. This review compiles the most significant research on minerals and mineraloids in the brain, the related neurodegenerative diseases, and their relationship with urban pollution. Here, we describe the strong link between neurodegenerative diseases and the presence of biogenic and exogenous minerals and mineraloids. Additionally, we highlight the importance of medical mineralogy for investigating diseases related to such phases. Future research must focus on not only the mineralogical characterization of particles in the brain but also the alteration and transformation of these particles in specific media and different locations in human brain cells and tissues. Further studies should attempt to perform nanoscale to atomic-scale characterization of the structure, the surface, the valence state, and the electrical and magnetic fields of the particles of interest.
- Article
(3779 KB) - Full-text XML
- BibTeX
- EndNote
Biogenic mineralization occurs in many organisms, including prokaryotes, plants, and animals. Magnetotactic bacteria, for example, use biogenic magnetite (Fe3O4) nanocrystals for geomagnetic orientation (Frankel et al., 1979; Bazylinski et al., 2013). In the plant kingdom, crystalline Ca-oxalates are very common (as phytoliths) and play a key role in carbon storage (Stephens, 2012; Trouvé et al., 2023). Similarly, silica phytoliths are biominerals that are deposited in intracellular and extracellular plant structures (Piperno, 2006; Vigliaturo et al., 2019). In animals, the precipitation of Ca-carbonate and Ca-phosphate biominerals is necessary for the development of structures such as shells, bones, cartilage, and teeth (Boskey, 2007; Duer et al., 2009). In addition to carbonate and phosphate minerals, studies have identified ferrimagnetic material in the tissues of animals such as chitons, bees, pigeons, and salmon (Gould et al., 1978; Kirschvink et al., 1985; Mann et al., 1988; Hanzlik et al., 2000; Pósfai and Dunin-Borkowski, 2009). The function of ferrimagnetic particles ranges from geomagnetic navigation (for magnetosensitive species), tooth hardening, Fe storage, and possibly memory (Lowenstam, 1962; Gould et al., 1978; Walcott et al., 1979; Mann et al., 1988; Chang and Kirschvink, 1989; Banaclocha, 2002; Zuddas et al., 2013; Krichen et al., 2017; Alfsen et al., 2018). A major scientific breakthrough was made when researchers discovered the presence of magnetite nanocrystals in a variety of human brain tissues (Kirschvink et al., 1992).
In the human brain, biomineralization is not always beneficial for physiological processes and functions. It has been suggested, for example, that the ability of eukaryotes, including humans, to synthesize biogenic magnetite nanoparticles (NPs) may be a latent, plesiomorphic trait inherited from magnetotactic bacteria (Shubin et al., 2009; Bellinger et al., 2022). Even though biogenic minerals may be crucial for healthy human brain functions, other biomineralization processes can contribute to devastating neurodegenerative conditions. Pathological mineralization, i.e., abnormal growth and accumulation of minerals inside soft tissues, can be toxic and can trigger an inflammatory response, which may lead to different diseases and, eventually, death. Virtually every soft tissue in humans might be subject to pathological mineralization (Tsolaki and Bertazzo, 2019). For instance, calcification in different regions of the brain has been linked to Fahr's disease, meningioma, and Parkinson's disease (PD) (Tsolaki and Bertazzo, 2019). Similarly, studies show a link between minerals and several neurological disorders (e.g., Fe- and Li-containing minerals). For example, lithium carbonate (Li2CO3) is commonly used for the treatment of bipolar disorder (Netto and Phutane, 2012; May et al., 2015), but an excessive exposure to Li can cause reversible and irreversible consequences, such as dementia, tremor, hyperreflexia, nystagmus, ataxia, and other brainstem-related illnesses, as well as complications in other organs (Verdoux and Bourgeois, 1991; Järup, 2003; Tchounwou et al., 2012; Jaishankar et al., 2014; Ott et al., 2016). Moreover, both elemental Fe and Fe-oxide minerals are frequently observed at different scales in different regions of the human brain and may contribute to the decreased cognitive function associated with neurodegenerative disorders such as PD and Alzheimer's disease (AD) (Ward et al., 2014).
In addition to endogenous minerals, exogenous phases (e.g., magnetite NPs) have been found in the human brain (Maher et al., 2016) after exposure to particulate matter (PM) in the urban environment. Once these particles and their metal constituents enter the body, they may interact with cells or body fluids. For instance, some particles can translocate from the lungs to other parts of the body, such as the nervous system or the liver. Alternatively, minerals can enter the brain directly through the olfactory bulb (Maher et al., 2016; Gonet and Maher, 2019), subsequently causing inflammation or oxidative stress in target tissues (Ward et al., 2014). Fine PM, often associated with transition and post-transition metals (e.g., lead in soils), can be particularly toxic to humans and can cause devastating neurological effects (O'Shea et al., 2021b). Global urbanization trends have led to a greater risk of exposure to PM for urban populations (Chen et al., 2014; United Nations Department of Economic and Social Affairs, Population Division, 2019; Zhan et al., 2023), and thus, organ-specific mineralogy has become an essential component of interdisciplinary science.
The characterization of both biogenic and exogenous minerals and mineraloids in the human brain remains largely unexplored. Nevertheless, some of these NPs may pose health risks. Addressing this gap is crucial, since many human activities lead to increasing concentrations of mineral and mineraloid particles in the atmosphere and the surface environments of Earth (Gieré and Querol, 2010; Grobéty et al., 2010; Dietrich et al., 2018, 2021; O'Shea et al., 2021a; Caballero-Gómez et al., 2022; Dietrich et al., 2022). According to several studies, exogenous particles with diameters smaller than 10 µm can be inhaled and may accumulate in the brain if smaller than 200 nm (Calderón-Garcidueñas et al., 2016; Maher et al., 2016). The link between the presence of excess reactive oxygen species (ROS) generating solid NPs in the brain and neurodegenerative diseases is clear (Casanova and Araque, 2003; Tsolaki and Bertazzo, 2019). However, the physicochemical identity of these particles and the mechanisms by which they interact with the host tissues causing neurodegeneration remain poorly understood (Tsolaki and Bertazzo, 2019). To better understand this relationship, it is critical to study both biogenic (pathogenic and non-pathogenic) and exogenous solid particles in the human brain.
In this review, we compile recent findings on three aspects of minerals and mineraloids occurring in the human brain, namely (1) minerals that naturally precipitate in the human brain, i.e., biogenic phases; (2) the contribution of air pollution to the presence of exogenous phases inside the human brain; and (3) the possible relationship between several diseases and the overexposure and subsequent accumulation of certain minerals and mineraloids inside the human brain. Specifically, we will highlight the presence and physicochemical characteristics of minerals and mineraloids that tend to generate ROS, which might be directly related to an inflammatory response of the body, toxicity, and some diseases. Moreover, we will discuss suitable methods for mineral characterization. To provide further information to the reader, we have collected relevant papers on minerals and mineraloids in the human brain (Table A1), and metals associated with Fe-oxides occurring in the urban environment (Table A2).
This brief summary on human brain anatomy is provided to give context about where minerals and mineraloids of interest have been observed in the brain and how they may interact with the surrounding biochemical environment.
The central nervous system (CNS) is divided into two main portions visible to the naked eye, namely the white matter and the gray matter. The white matter is composed mainly of lipids, whereas the gray matter comprises the cortex, the nuclei of neurons, and the ganglia (Kreutzer et al., 2011). The brain is the primary component of the CNS in humans (Clark et al., 2010) and consists of the cerebrum, cerebellum, and brainstem (Fig. 1). The cerebrum and cerebellum are surrounded by the cortex (Tsolaki, 2020).
The cerebrum is divided into several lobes and subcortical structures, including the hippocampus. The cerebral cortex is a 2–4.5 mm thick mass of gelatinous material (Fischl and Dale, 2000), which is split into two portions (left and right) and four sets of primary lobes (frontal, parietal, temporal, and occipital lobes; see Fig. 1) for a total of eight lobes. Each lobe is dedicated to specific functions (Jones, 2017): the largest primary lobe, the frontal lobe (Fig. 1), is located in the front of the brain and has various functions ranging from motor control to language generation; the parietal lobe is responsible for proprioception; the temporal lobe is responsible for hearing and understanding of language; and the occipital lobe is responsible for visual computation. The cerebellum (Fig. 1) is responsible for numerous functions, including cognition, emotion, sensory reception, attention, threat, and pleasure (Strick et al., 2009). The brainstem links the cerebrum and the cerebellum to the spinal cord, and it is fundamental for the organism's survival.
In the inner part of the cerebrum, there is a complex of five subcortical nuclei called the basal ganglia (Fig. 2). The basal ganglia include (1) the putamen and (2) the caudate nucleus, which together form the striatum; (3) the globus pallidus; (4) the substantia nigra; and (5) the subthalamic nucleus. The primary function of the basal ganglia is to select and carry out intentional actions in response to both internal and external stimuli (Simonyan, 2019). The basal ganglia are primarily responsible for facilitating voluntary movements while simultaneously inhibiting movements that compete with or interfere with them (Mink, 1996; Redgrave et al., 1999; Simonyan, 2019). Their involvement is also connected to the regulation of many other intricate non-motor functions, including working memory, language, emotions, and procedural learning (Simonyan, 2019). Closely related to the basal ganglia are the amygdala (Fig. 2) and the thalamus (Fig. 2), both part of the limbic system, which is involved in short-term memory, the sense of smell, and emotions (Friedman and Chou, 2007). The amygdala is a group of nuclei located in the midline, along with the putamen and caudate nucleus (Friedman and Chou, 2007).
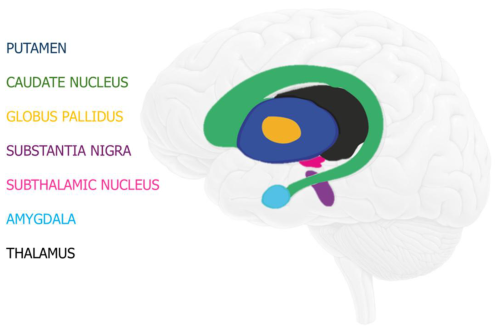
Figure 2Scheme showing the basal ganglia, as well as the amygdala and the thalamus. Image modified from Andruşca (2023).
The environment of the CNS is separated from that of the peripheral circulation by the blood–brain barrier (BBB). The BBB maintains a homeostatic environment in which CNS components can function without disruption from other body functions (Dotiwala et al., 2023). The BBB is a selective semipermeable membrane, located between the blood and the interstitium of the brain. The BBB allows cerebral blood vessels to control molecule and ion movement between the blood and the brain tissues (Sanders et al., 2009; Dotiwala et al., 2023; Gawdi et al., 2023). To achieve this function, the BBB is composed of tight junctions and enzymes that provide physical and metabolic barriers, respectively (Correale and Villa, 2009).
For decades, it has been known that the human brain contains various metals in different speciations and that these metals are unevenly distributed. For example, Duflou et al. (1989) observed using particle-induced X-ray emission (PIXE) that the elemental concentrations of K, Fe, Cu, Zn, Se, and Rb were higher in gray matter than in white matter. Moreover, Mn, Fe, and Cu were present in high concentrations (as elements) in basal ganglia (Fig. 2), and no systematic differences in terms of elemental concentration between the two hemispheres were noticed (Duflou et al., 1989).
While concentrations of these elements in the brain have been thoroughly studied, very little information has been gathered regarding the speciation of these elements in the form of solid phases, such as minerals and mineraloids. Moreover, little to no data have been collected to investigate the structural and ultra-structural localization of minerals with respect to brain cells, tissues, and compartments (Tsolaki, 2020).
3.1 Minerals and mineraloids containing Fe occurring in the human brain
Iron is vitally important for several biological processes that take place inside the human brain, mainly because it is an essential redox-active cofactor, which is involved in key activities like respiration and redox sensing through Fe–S clusters, heme, and mononuclear Fe sites that regulate protein activity (Connor et al., 2001; Outten and Theil, 2009; Tisato et al., 2009). For instance, Fe is an essential component of enzymes that contribute to reactions (Everett et al., 2018). Both Fe and Cu are used extensively in multiple physiological processes that are strictly regulated and necessary for the high energy demand of the human brain (Connor et al., 2001; Scheiber et al., 2014). Iron is frequently needed for several neuronal functions (Connor et al., 2001), such as energy production, nerve impulse transduction, and neurotransmitter synthesis (Connor et al., 2001; Zecca et al., 2004). The concentration of Fe ions is physiologically regulated by redox reactions, such as the ferroxidase function of ferritin (Everett et al., 2021), a complex ferrihydrite–phosphate–protein shell (Chasteen and Harrison, 1999; Connor et al., 2001).
The first observations of fine granular deposits of Fe in human brain tissue, specifically in the gray matter and brainstem (Fig. 1), were made by Spatz (1922). Since these observations were obtained by optical microscopy, it was not possible to identify the mineral species in the observed Fe deposits, nor to determine their physicochemical characteristics. Similar Fe deposits were later detected in the walls of human blood vessels, also by means of optical microscopy (Hadfield, 1929). A few years later, Gellerstend (1933) observed Fe deposits in the occipital cortexes of individuals over 65 years old without mental or neurodegenerative diseases. While performing experiments on the brains of adult humans and children, Tingey (1937, 1938) extracted non-heme Fe (i.e., elemental Fe that does not bind to a porphyrin ring) using pyrophosphate and observed with optical microscopy that lower amounts of Fe were present in the brains of children compared to those of adults. Cumings (1948) quantified the amount of elemental Fe in each anatomical part of six human brains and discovered that the highest amount of elemental Fe was systematically found in the globus pallidus, caudate nucleus, thalamus (Fig. 2), and the cortical gray and white matter. Mahler (1955) discovered that brain mitochondria as well as microsomes contain a considerable amount of non-heme elemental Fe. The reported increase in the concentration of non-heme elemental Fe in the brain during development was attributed to changes in the cell's enzyme systems (Hallgren and Sourander, 1958; Ward et al., 2014). Moreover, Hallgren and Sourander's study (1958) revealed that numerous portions of the cerebrum contain high concentrations of elemental Fe, such as the dentate nucleus, the basal ganglia, and a portion of the midbrain. All these studies, however, merely determined the presence of Fe deposits, without investigating the mineralogical species and the physicochemical state of the phases or possibly amorphous inorganic compounds present in the biological samples. This drawback is a result of having restricted the investigations to optical microscopy and bench chemistry techniques, making it impossible to determine the mineralogical state of the Fe deposits detected.
To date, the two main ferrimagnetic minerals that have been identified in the human brain are magnetite (Fig. 3a) and maghemite (Fig. 3b) (Pósfai and Dunin-Borkowski, 2009). Magnetite (Fe2+FeO4) is the most common biogenic magnetic mineral found in many living organisms (Kirschvink, 1992). Magnetite has a cubic structure (Bragg, 1915), in which Fe3+ is coordinated tetrahedrally with O, whereas both Fe3+ and Fe2+ are coordinated octahedrally with O (Fleet, 1981; Kozlenko et al., 2019). Natural magnetite at room temperature has structural defects, such as an interstitial-vacancy couple similar to ferrous oxide (FeO) (Wechsler et al., 1984). Maghemite (γ–Fe2O3) has the same structure as magnetite, except that all Fe is trivalent and one-sixth of the octahedral sites have random vacancies (Labarta et al., 2005). Both magnetite and maghemite are recognized as members of the cubic spinel group (Pecharroman et al., 1995; Shokrollahi, 2017).
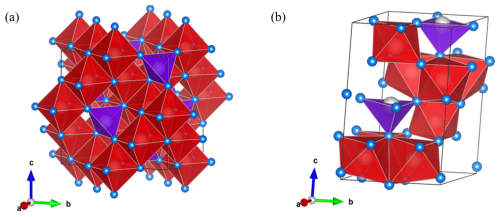
Figure 3Fe-oxide mineral phases in the human brain: (a) magnetite (COD code 9007644) and (b) ferrihydrite (COD code 9011571), created with VESTA. O – blue; Fe – gray; tetrahedra – purple; and octahedra – red. The unit cell is shown in black.
Due to their location, spatial orientation, and potential for signal transmission, biogenic magnetite NPs may play an important role in several cerebral functions. As a speculative hypothesis, magnetite may contribute to systems that control short-term memory (e.g., a prion–magnetite tandem mechanism for memory storage), creativity, imagination, and thinking (Zuddas et al., 2013; Alfsen et al., 2018). Biogenic magnetite has been found in the hippocampus, the neocortex, and the amygdala (see Fig. 2), all of which are brain regions that are related to short-term memory (Kirschvink et al., 1992; Dobson and Grassi, 1996; Dobson, 2001; Shapiro, 2001; Brem et al., 2006). Theories suggest that inside the phospholipid membrane of human neuronal cells are magneto-sensitive ionic channels (Kirschvink and Gould, 1981; Kirschvink, 1992; Kirschvink et al., 1992; Goychuk, 2018). The coexistence of both magnetite and these ion channels in the brain may indicate a link to neuronal activation (Kirschvink and Hagadorn 2000; Cadiou and McNaughton, 2010). Moreover, sensorial neurons of the peripheral nervous system present mechanosensitive ion channels, which are responsible for our sense of touch, equilibrium, and pain (Coste et al., 2010; Delmas et al., 2011). These mechanosensitive channels may open when there is tension in the membrane (Christiansen et al., 2019). Ferrimagnetic NPs, such as magnetite, may influence these ion channels if the latter are in proximity (Hughes et al., 2007; Lee et al., 2014; Tay and di Carlo, 2017). Biogenic magnetite could be involved in the neural-activity-associated magnetic fields (NAAMFs) generated in neocortical mini columns, as suggested by Banaclocha (2002). Indeed, biogenic magnetite may be crucial for the transmission of magnetic signals generated inside the neocortex (Banaclocha et al., 2010). Biogenic magnetite appears to be uniformly distributed in the membranes of neurons and astrocytes (star-shaped glial cells in the CNS), which produce the strongest magnetic fields in the brain (Brem et al., 2006). The results of a study by Banaclocha et al. (2010) suggest that NAAMFs may regulate the distribution and organization of biogenic magnetite NPs in neuronal membranes, which would mean that there is a nonrandom distribution of these crystals on neuronal surfaces. This specific distribution of magnetite on neuronal surfaces could play an important role in the induction of static 3D magnetic constructs in neocortical astrocytes. Altogether, the distribution of biogenic magnetite may allow for long-term data storage in the neocortical astrocyte network (Banaclocha et al., 2010). Biogenic magnetite crystals in the human brain should interact more intensively with the Earth's magnetic field than other biogenic, diamagnetic, or paramagnetic substances, such as deoxyhemoglobin, ferritin, and hemosiderine (Zuddas et al., 2013). On the other hand, the interaction between the Earth's magnetic field and both biogenic magnetite and other biogenic diamagnetic or paramagnetic compounds is somewhat limited due to the shielding effect of the skull (Zuddas et al., 2013).
In general, biogenic magnetite ranges in size from tens to hundreds of nanometers (nm), and the crystals can be divided into three main dimensional groups, which are related to their magnetic properties (Pósfai and Dunin-Borkowski, 2009; Li et al., 2017). The first group is identified as single-magnetite NPs, which are smaller than 30 nm and are superparamagnetic (SPM) at room temperature (i.e., thermal motion constantly shifts the orientations in which the crystals are magnetized) (Huber, 2005; Pósfai and Dunin-Borkowski, 2009; Li et al., 2017). Biogenic magnetite in this size range can be found, for instance, in humans (Quintana et al., 2004). The second group is made up of magnetic single-domain (SD) grains with diameters between approximately 30 and 120 nm. Magnetite particles in this group have been found in a variety of organisms, including bacteria and fish (Pósfai and Dunin-Borkowski, 2009; Li et al., 2017). The third group refers to isolated magnetite NPs, which are larger than about 120 nm and which are identified as “magnetic multi-domains” (MD) (Pósfai and Dunin-Borkowski, 2009; Li et al., 2017).
Despite the general lack of research focused on the mineralogy of the human brain, there are some pivotal studies on this topic. Magnetite NPs in the SPM and magnetic SD size ranges have been found in the human brain (Kirschvink et al., 1992; Quintana et al., 2004). Using isothermal remanent magnetization (IRM), Kirschvink et al. (1992) discovered the presence of magnetite and other ferrimagnetic minerals in the cerebral cortex, cerebellum, and meninges (membranes surrounding the brain and spinal cord). These researchers further identified the presence of magnetite–maghemite crystals of approximately 30 nm in size using high-resolution transmission electron microscopy (HR-TEM) and selected-area electron diffraction (SAED). According to Kirschvink et al. (1992), in 1 g of the human brain there are around 5 to 100 million biogenic magnetite NPs, exhibiting diameters ranging from 10 to 200 nm and having a bimodal distribution. Magnetite NPs in the human brain are located intracellularly, tied to the membrane, and aggregated in chains (Kirschvink et al., 1992; Quintana et al., 2004; Collingwood et al., 2008). The magnetite found by Kirschvink et al. (1992) seemed to be mainly a magnetic SD (Zuddas et al., 2013). Lattice images generated by SAED and HR-TEM examination combined with chemical analysis by energy-dispersive X-ray spectroscopy (EDXS) revealed euhedral crystals, suggesting their biogenic origin (Mann et al., 1988) since only magnetite that grows in situ (e.g., the human brain) can display euhedral habitus (Mann et al., 1988). On the other hand, Collingwood et al. (2008) used TEM dark-field images to analyze the size distribution of SPM-sized magnetite particles in amyloid plaques and suggested that these NPs may have originated from a ferritin precursor.
Other than biogenic magnetite, non-heme Fe is stored in the human brain in the form of approximately 8 nm NPs of ferrihydrite (FeO14(OH)2) (Fig. 3b), a hydrous ferric oxide surrounded by a protein shell. Beside ferrihydrite, inside this protein shell known as ferritin, there are different percentages of phosphate (Treffry et al., 1987; Harrison and Arosio, 1996; Michel et al., 2007). Unlike magnetite, ferrihydrite is anti-ferromagnetic (Gilles et al., 2002). Due to the hydration and the multiple lattice vacancies, ferrihydrite is usually the less stable precursor to mineral phases such as hematite (Fe2O3), which is a canted anti-ferromagnet, i.e., a phase with a very weak magnetic moment (Towe and Bradley, 1967; Eggleton and Fitzpatrick, 1988). Some theories suggest that the ferrihydrite inside ferritin could be the precursor to biogenic magnetite NPs (Plascencia-Villa et al., 2016; Gorobets et al., 2017), but there is no evidence for a direct transition from ferrihydrite to magnetite in the human brain (Gálvez et al., 2008; Quintana and Gutiérrez, 2010; Friedman et al., 2011; Gorobets et al., 2017).
3.2 Minerals and mineraloids containing Al and Si occurring in the human brain
Aluminum is a nonessential toxic element (WHO, 2011), and it has been detected mostly inside the gray matter (approximately 0.40 µg g−1 dry weight) via laser microprobe mass analysis (LAMMA) and inductively coupled plasma mass spectroscopy (ICP-MS) (Freundlich, 1985; Bush et al., 1995; Roider and Drasch, 1999; Reusche et al., 2001; Exley and House, 2010). Specifically, the presence of Al was reported in several regions of the human brain. Aluminum concentrations of up to approximately 50 µg mL−1 (dry weight) were detected in the BBB (Exley et al., 2006), as well as in the interstitial fluid, non-neuronal cells (i.e., astrocytes, oligodendrocytes, microglia, mononuclear migratory cells), and neurons. Moreover, studies conducted by LAMMA (Bush et al., 1995; Bouras et al., 1996; Reusche et al., 2001), X-ray spectroscopy (Shirabe et al., 2002), and fluorescence microscopy (Exley and Mold, 2020) demonstrated that amyloid plaques, neurofibrillary tangles, Lewy bodies, and lipofuscin can act as sinks for the deposition of Al (Exley and House, 2010). Consequently, it was theorized that Al deposited in neurofibrillary tangles could be an etiologic agent for neurodegenerative disorders (Perl and Moalem, 2006). This theory is consistent with the findings of Candy et al. (1986), who reported NPs recognized as aluminosilicates in the cores of amyloid plaques occurring in the brain. The identification of these crystalline phases, however, was achieved using SEM-EDXS, and thus, there is no information on the crystal structure, d spacing, or the detailed morphometry of these compounds. High-resolution investigation techniques such as high-resolution scanning/transmission electron microscopy (HR-S/TEM), EDXS, dual-electron energy loss spectroscopy (EELS), and 4D-STEM are needed to investigate the crystallochemical identity of these NPs. The presence of Al and Si in the cores of amyloid plaques was investigated by multiple research teams, but the results were contradictory, and no significant concentration of Al and Si was found through LAMMA in the cores of amyloid plaque in cases of investigations into AD (Stern et al., 1986; Landsberg et al., 1992).
To the best of our knowledge, no other recent study demonstrated the presence of specific silicates in the human brain, either exogenous or biogenic. Nevertheless, exogenous silicate NPs could potentially enter the human brain since manufactured Si phases are ubiquitous in urban settings (e.g., amorphous silica, crystalline silica, glass beads in traffic paint) (Reuzel et al., 1991; Lewinson et al., 1994; ATSDR, 2019; O'Shea et al., 2021c; Burghardt et al., 2022).
3.3 Minerals and mineraloids containing Cr occurring in the human brain
The most common oxidation states of Cr in the near-surface environment are Cr3+ and Cr6+. It is common knowledge that Cr3+ is an important micronutrient, which plays a role in glucose metabolism (Reeder et al., 2006). On the other hand, Cr6+ is a carcinogen, is linked to both acute and chronic health impacts, and is a contact allergen (EPA, 1998; ATSDR, 2000). Chromium exposure occurs mostly through drinking water, unintentional ingestion of soil, and inhalation of mineral dust that contains Cr (O'Shea et al., 2021c). After Cr assimilation, Cr6+ is more bioavailable than Cr3+ (ATSDR, 2000; Reeder et al., 2006). Unlike Cr3+, Cr6+ may easily pass through the membrane of cells, such as red blood cells, and reach all organs, including the brain (ATSDR, 2000; Reeder et al., 2006).
To the best of our knowledge, the presence of biogenic Cr minerals in the human brain has never been reported. However, since anthropogenic Cr-bearing NPs are common in urban PM (Adachi and Tainosho, 2004; Sanderson et al., 2014; O'Shea et al., 2021c), they may pose a risk to human health, as they are sufficiently small to pass through the BBB (Kreuter et al., 2002). O'Shea et al. (2021c) studied the possible physicochemical interactions between simulated gastrointestinal fluids and Si-coated crocoite (PbCrO4) in yellow traffic paint, but no research was carried out with simulated brain fluids. An experimental setup similar to the one described in O'Shea et al. (2021c) could be easily adapted to explore the interaction between crocoite and brain fluids or neuron cytoplasm. Nevertheless, some studies were conducted to investigate possible neurotoxic effects in animal models after chronic exposure to exogenous Cr-oxide. Fatima et al. (2017) focused on biochemical and histological changes in the kidneys and brains of rats exposed to Cr2O3 NPs. At the end of the experiment, the researchers detected an increased production of ROS, higher malondialdehyde concentration, and decreased superoxide dismutase and glutathione levels, indicating cellular injury and functional impairment. It can be deduced that exposure to Cr-oxide NPs may have similar consequences in humans, but no studies have been performed on humans, nor was Cr detected in thin sections of the human brain, possibly due to instrumental limitations (e.g., insufficient spatial resolution).
3.4 Minerals and mineraloids containing Cu, Ni, Co, and Pt occurring in the human brain
Copper phases containing bivalent (Cu2+), monovalent (Cu+), and metallic (Cu0) Cu have been detected in amyloid plaques through synchrotron-based scanning transmission X-ray microscopy (STXM) (Everett et al., 2021). Using HR-TEM–EDXS, Maher et al. (2016) observed the presence of other heavy metals (e.g., Ni, Co, Pt) within particles retrieved from the frontal cortex of 37 human brains. NPs have high specific surface areas, potentially enhancing the surface adsorption of some heavy-metal cations (Halsall et al., 2008; Maher, 2019).
3.5 Minerals and mineraloids containing P and Ca occurring in the human brain
Calcification in the human brain is a well-documented phenomenon, involving Ca deposits in various regions of the brain, ranging from perivascular areas to the basal ganglia complex (Mahy et al., 1999; Loeb et al., 2006; Maetzler et al., 2010; Monfrini et al., 2023). Brain calcification has been associated with several neurological disorders, including Fahr's disease, AD, PD, and the normal aging process (Mahy et al., 1999; Loeb et al., 2006; Monfrini et al., 2023). Small crystalline particles primarily composed of Ca-carbonate have been detected in the pineal gland, an organ located in the anatomical center of the human brain (Baconnier et al., 2002). These particles resemble calcite crystals and exhibit piezoelectricity. The growth of these crystals could be regulated by acidic glycoproteins, similar to those used during biomineralization processes in other organisms such as sea urchins and sponges (Aizenberg et al., 1997; Baconnier et al., 2002).
Mahy et al. (1999) examined basal ganglia tissue using TEM-EDXS and concluded that in the basal ganglia there are depositions of Ca and P compounds that correspond to apatite. The ratio and particle size (>10 µm) of these Ca and P compounds further support the hypothesis that these deposits are biogenic (Mahy et al., 1999). Mahy et al. (1995) concluded that the Ca and P biogenic precipitation in the basal ganglia could be part of a compensatory response to excitotoxic neurodegeneration.
Magnetotaxis is a mechanism used by magnetotactic bacteria to align themselves to Earth's magnetic field (Pósfai and Dunin-Borkowski, 2009). Magnetotactic bacteria accumulate Fe from the surrounding environment and crystallize ferrimagnetic particles within the cell (Pósfai and Dunin-Borkowski, 2009). Arrangement of these magnetite crystals in a line from head to tail gives the cell a dipole moment, allowing for its passive orientation followed by active motility by flagella along magnetic field lines (Gould and Kirschvink 1978; Blakemore, 1982).
The size, shape, and spatial orientation of synthesized magnetite must be tightly regulated in magnetotactic bacteria (Pósfai and Dunin-Borkowski, 2009) by biologically controlled mineralization (BCM) (Staicu et al., 2021) for the crystal to perform a specific function. Magnetotactic bacteria accumulate and crystallize magnetite nanocrystals inside specific organelles called “magnetosomes” (Gorby et al., 1988; Vali et al., 1991; Faivre and Schüler, 2008). Magnetosomes are subcellular structures (an ensemble of the phospholipid membrane vesicle and the magnetic nanocrystal inside) encoded by about 30 genes, most of which are located on a magnetosome genomic island (Fukuda et al., 2006). Firlar et al. (2016) used high-angle annular dark field (HAADF) STEM with EDXS imaging, SAED, and STEM-EELS to investigate the sizes and crystalline structures of particles created by magnetosomes as well as the chemical environment of Fe and O in the magnetosomes. Firlar et al. (2016) discovered that the increase in particle size of the biomineral phase over time is closely connected with biomineralization of NPs. The initially generated magnetosomes are amorphous ferric hydroxides smaller than 10 nm in size. These are then converted to crystalline two-line ferrihydrite in the transition stage (i.e., 10–15 nm) and finally to magnetite, with a remnant of two-line ferrihydrite. HR-TEM observations of bacterial biogenic magnetite revealed stoichiometric, perfectly crystalline NPs (with diameters between 35 and 50 nm) that were consistent in shape, uniformly magnetized, usually elongated along the [111] direction, and contained a relatively low number of impurities compared to abiotically grown crystalline magnetite (Butler and Banejree, 1975; Mann et al., 1984a, b; Mann, 1985; Vali et al., 1991; Diaz-Ricci and Kirschvink, 1992). Even though magnetosome magnetite is usually chemically pure, Mo and Sn have significant affinities for magnetite synthesized by magnetotactic bacteria, most likely due to the metabolic relevance of these elements, which must be absorbed into the intracellular medium to sustain the bacterial metabolic activity (Amor et al., 2020). Clearly, HR-TEM techniques are needed to investigate nanoscale to atomic-scale NPs in organisms (in this case, magnetotactic bacteria) (Firlar et al., 2016). The same procedures can be applied to tissue sections of the human brain.
As in the case of magnetotactic bacteria, some theories suggest that several migratory vertebrates, including turtles (Pósfai and Dunin-Borkowski, 2009), birds (Hanzlik et al., 2000), and salmon (Mann et al., 1988), may synthesize ferrimagnetic NPs in their brains. These theories, however, are also criticized by other studies (Treiber et al., 2012; Edelman et al., 2014; Mouritsen, 2018) that did not identify any biogenic ferrimagnetic NPs in brain tissues of migratory birds. However, magnetosome gene homologues (MGHs), which comprise roughly 11 genes, are universally present in eukaryotes (Bellinger et al., 2022). MGHs are homologous to the magnetotactic bacteria genes deputed to magnetite biomineralization (Bellinger et al., 2022). These MGHs include the MamE gene (responsible for protein storage in magnetotactic bacteria) and the MamH and MamN genes (both dedicated to magnetite crystallization in magnetotactic bacteria). The MamH and MamN genes, for instance, are members of the mamAB operon (Lohße et al., 2011; Murat et al., 2010). There is no evidence for the presence of MGHs from the three other magnetosome-associated operon-like gene clusters (mamGFDC, mamXY, and mms6).
The presence in the human genotype of only one homolog gene for transcribing the protein designated for the synthesis of biogenic magnetite (Gorobets et al., 2013, 2014; Medviediev et al., 2017) implies that during the synthesis of biogenic NPs, human cells are not able to completely control the dimensions, shape, crystallinity, and chemical composition of the mineral phases since humans do not possess all MGH operons assigned to control these features (Gorobets et al., 2014). Bellinger et al. (2022) hypothesized that magnetite biomineralization could represent profound homology, a latent, plesiomorphic ability (genetic and cellular) to synthesize biogenic magnetite NPs (Shubin et al., 2009) for magnetoreception purposes in eukaryotes, including humans (Vali et al., 1991; Kirschvink and Hagadorn, 2000). Indeed, biological functions such as hardening certain organs (Lowenstam, 1962) or storing Fe (Pósfai and Dunin-Borkowski, 2009) as a source of chemical energy to sustain a cell's metabolism (Byrne et al., 2015) are largely independent from the crystals' physical characteristics.
The term “atmospheric PM” refers to solid and liquid particles that are suspended in the air and are derived from natural or anthropogenic processes (Gieré and Querol, 2010; WHO, 2021). As PM can be emitted from various sources, including industrial facilities, power plants, incinerators, cars, volcanoes, and fires, it varies in composition and size (Morabet, 2018). The World Health Organization (WHO) implemented the air quality guidelines (AQGs) and estimated reference levels (RLs), taking into consideration sizes of 10 µm or less, which are inhalable by humans (WHO, 2021). PM is then categorized into two size groups: PM10 is particles with an aerodynamic diameter of 10 µm or less, whereas PM2.5 is particles with an aerodynamic diameter of 2.5 µm or less. In the scientific community, an additional size category – ultrafine particles (UFPs) – has been defined as particles with an aerodynamic diameter of 0.1 µm or less (Li et al., 2016; Kwon et al., 2020; WHO, 2021).
The main pathway by which people are exposed to airborne contaminants is through inhalation (Leikauf et al., 2020). After initial inhalation, particles are deposited depending on their size (Fig. 4). For example, PM10 is sequestered in the upper airway or conducting lower airways, whereas PM2.5 can reach the bronchioles and alveoli (Oberdörster, 1993; Kreyling et al., 1999; Möller et al., 2004). UFPs, on the other hand, can reach several other areas of the body, including the brain. The uptake of UFPs by nerves in the upper and lower respiratory tract was already demonstrated decades ago (Hunter and Dey, 1998; Hunter and Undem, 1999). Upon inhalation, UFPs pass through the nasal canal and can directly reach the olfactory nerve (and the related olfactory bulb), as well as the trigeminal, facial, and glossopharyngeal nerves (Calderón-Garcidueñas et al., 2019). The nasal mucosa contains sensory nerve endings of the ophthalmic and maxillary branches of the trigeminus nerve: these can represent an alternative translocation pathway for solid UFPs (Hunter and Dey, 1998; Schaefer et al., 2002). The olfactory nerve act as a direct link between the respiratory tract and the CNS (Calderón-Garcidueñas et al., 2019, 2020). This intersection between UFPs, the upper respiratory system, and the brain was demonstrated by Calderón-Garcidueñas et al. (2016), who found deposits of UFPs in the nose and portions of the brains of children living in metropolitan Mexico City. If not directly detected by the olfactory nerve, UFPs can easily migrate to the lower respiratory tract, reaching the alveoli, where they interact with the alveolar macrophages. Most particles are sequestered by macrophages (Takenaka et al., 2001). All particles that have been sequestered by macrophages are englobed in the mucus layer and subsequently transported to the oropharynx and hence swallowed into the gastro-intestinal tract (Oberdörster, 1993; Semmler-Behnke et al., 2007; Beamish et al., 2011). However, macrophages that interact with PM2.5 and UFPs can undergo a phenomenon known as “frustrated phagocytosis”, which means that these cells may not entirely digest the particles detected and may even shrink, making it easier for particles to enter the bloodstream. This phenomenon depends on the size and shape of the particles (Honarpisheh et al., 2017; Francis et al., 2022; Wieland et al., 2022). On the other hand, UFPs may not be detected and intercepted by macrophages and may pass through the air–blood barrier (ABB), thus entering the blood circulation. The ABB is composed of the alveolar epithelium, the fused basement membrane, and the capillary endothelium (MESH NCBI, 2024). The ABB separates capillary blood from alveolar air, permitting pulmonary gas exchange (Su et al., 2020; MESH NCBI, 2024). Once in the blood circulation, UFPs can pass the BBB, especially when it is compromised, and penetrate the neurovascular unit (NVU). If protein- or lipid-coated, UFPs have an increased chance of entering the CNS and reaching the brain (Kreuter et al., 2002). Maher et al. (2016) were the first to highlight the presence of spherical magnetite NPs (up to 150 nm across) in the frontal cortex (Fig. 1) of 37 human brains, using HR-TEM-EDXS and a superconducting quantum interference device for magnetic analysis (SQUID magnetometry). The spherical shape and smooth surface texture of these particles indicate formation at high temperatures (i.e., combustion processes – Gieré et al., 2003). Furthermore, their spherical morphology is not consistent with the euhedral morphology of biogenic magnetite (Kirschvink et al., 1992). The surface texture, size, and size distribution of these spherical magnetite NPs described are totally distinct compared to the biogenic magnetite described in previous studies (Kirschvink et al., 1992; Schultheiss-Grassi, 1999; Quintana et al., 2004), suggesting that they originate from anthropogenic sources (Maher et al., 2016).
Another possible pathway by which PM can reach the blood circulation and consequently the brain is through ingestion. According to several studies (Oberdörster, 1993; European Commission, 2002; Kampa and Castanas, 2007; Beamish et al., 2011; De Brouwere et al., 2012; Salim et al., 2013; Calderón-Garcidueñas et al., 2020; Hammond et al., 2021), PM can be directly ingested by consuming contaminated food. An individual is thought to consume 1012–1014 microparticles each day (Lomer et al., 2002, 2004). These direct and indirect (i.e., through the macrophage mucus mentioned above) modes of exposure can collectively expose the digestive tract to considerable levels of PM (Oberdörster, 1993; Beamish et al., 2011; De Brouwere et al., 2012; Salim et al., 2013).
UFPs that easily reach the brain can be a good vector for other toxic elements previously suspended in the polluted air, as highlighted in Acosta et al. (2011). The presence of transition metals in the structure or adsorbed onto the surface of mineral particles could represent a potential risk to human health due to their physical and chemical properties, such as redox activity, magnetic behavior, and surface charge (Schoonen et al., 2006; Maher, 2019). The smaller size of particles is associated with a larger specific surface area, which has a major influence on the particles' cytotoxicity (Oberdörster, 2000; Donaldson et al., 2001). For example, the cytotoxicity of PM2.5 is largely attributed to heavy metals, which are a significant component of PM2.5 and can be bioaccumulated in the body (Fang et al., 2013). Kanapilly et al. (1982) found a difference in pulmonary deposition and retention between 20 and 100 nm particles of gallium oxide, whereby the smaller ones were cleared more rapidly by the lungs; an explanation for this could be that 20 nm particles are too small to be detected and hence phagocytized by macrophages, allowing them to translocate into the blood. According to earlier research, PM-bound metals may have a negative impact on human health (Lai et al., 2016). Despite the potential risks posed by the exposure to transition and post-transition metal-bearing NPs from urban road dust, little to no research has considered how nanoscale and sub-nanoscale particles enriched in heavy metals at or near their surface affect these interactions in a brain-simulating medium or in a real system. Without any doubt, having more information about the interaction of these particles at nanoscale resolution will provide useful contributions to the understanding of the mechanistic processes leading to toxicity and damage to the human brain. For this reason, the characterization of UFPs and the bulk-absorbed or surface-adsorbed heavy metals is necessary to predict their path to and through the human brain (Swift et al., 1992; Cheng et al., 1993).
Neurological disorders affect the body's autonomic, peripheral and central nervous systems (Farooqui, 2016). Worldwide, neurological disorders are the main cause of disability, and the second-most-frequent cause of death (Steinmetz et al., 2024). Enquiries conducted by the Global Burden of Disease (GBD) showed that since 1990, the number of deaths from neurological disorders increased by 39 %, while the disability-adjusted life-years (DALYs, i.e., the sum of years of life lost and years lived with disability) increased by 15 % (Feigin et al., 2019). In addition to their crucial cerebral functions, Al- and Si-containing NPs, Ca phosphates, and Fe minerals detected in the human brain have been linked to neurological disorders (Candy et al., 1986; Mahy et al., 1999; Hautot et al., 2003; Quintana et al., 2004; Loeb et al., 2006; Maetzler et al., 2010; Monfrini et al., 2023). Neurodegenerative diseases are a subset of neurological disorders characterized by the progressive degeneration of nerve cells (neurons), and they include AD, PD, and Huntington's disease (Gorell et al., 1997; Ballard et al., 2011; Everett et al., 2021). The most prevalent form of dementia is AD, followed by PD (Gorell et al., 1997; Parasrampuria et al., 2023; Martyr et al., 2024).
In general, several transition and post-transition metals have been found to be associated with multiple neurodegenerative diseases or detrimental conditions, whereby the effects are usually related to the ability of their host minerals to directly or indirectly produce ROS: firstly, some of the minerals that may reside in the human brain can release metal ions or adsorb metals or chemical compounds (e.g., polycyclic aromatic hydrocarbons), which can become electron donors and increase the rate of ROS formation; secondly, extremely reactive minerals or crystal-lattice defects may interact with carbon dioxide, water, or molecular oxygen to generate ROS; and lastly, exogenous insoluble minerals, which are ingested or internalized via the respiratory system, can cause cellular inflammation and produce secondary cellular ROS (Schoonen et al., 2006; Maher, 2019).
Amyloid plaques, extracellular deposits composed mainly of neural axons, amyloid beta (Aβ) proteins, and precipitated metal ions, are symptoms of neurodegenerative diseases, including AD (Yang et al., 2022). The presence of magnetite was only recently discovered in amyloid plaques (Plascencia-Villa et al., 2016; Telling et al., 2017; Everett et al., 2018) and may play an important role in neurodegeneration. Whether this role is part of the progression or the initiation of neurodegenerative disease is still unclear (Dobson, 2001). Elemental Cu and Zn are typically also bound by amyloid plaques (Lovell et al., 1998; Everett et al., 2021). The precipitation and deposition of Fe inside amyloid plaques may result from a possible perturbation in Fe homeostasis and dopamine metabolism (Connor et al., 2001). A perturbation of this sort can lead to excess Fe in brain cells (Ru et al., 2024). Goodman (1953) was the first to discover an abnormal accumulation of Fe in the brains of patients affected by AD. Other studies agree that there is a correlation between elevated Fe concentrations and neurodegenerative diseases (Beard et al., 1993; Smith et al., 1997). Increased levels of material containing Fe2+ may catalyze redox processes inside the human brain, which can cause the progression of neurodegenerative diseases (Hautot et al., 2003; Everett et al., 2018). Magnetite is thermodynamically more stable than other Fe phases, including Fe2+ ions, under the conditions found in the brain, so its correlation with AD pathogenesis is extremely important (Castellani et al., 2007). There is also a reported link between the formation of magnetite crystals in human brains and other neurodegenerative diseases (Dobson and Grassi, 1996; Dobson, 2001; Hautot et al., 2003). From these studies, researchers hypothesized that neurodegenerative diseases, such as AD, are linked to SPM magnetite particles since they are observed in amyloid plaques (Collingwood et al., 2008). Because Fe accumulation and associated oxidative stress have been shown to be early symptoms of AD (Nunomura et al., 2001), the presence of excessive quantities of redox-active Fe may be an important factor in triggering amyloid plaque aggregation and free-radical damage (Everett et al., 2018).
Hautot et al. (2003) used highly sensitive SQUID magnetometry to detect the presence of magnetite in human brain tissue and to distinguish it from the ferrihydrite NPs (which are encapsuled in the ferritin protein shell) (Massover, 1993; Chasteen and Harrison, 1999). The samples were retrieved from human brain tissues of both AD-affected patients and people who died with no known neurodegenerative diseases. The samples were then divided into two categories: one with a considerable amount of magnetite and the other containing little to no magnetite. The results obtained by Hautot et al. (2003) confirmed the expected dominance of ferrihydrite in the brains of both populations. However, brain samples with considerable concentrations of magnetite (between 1 and 7 µg of magnetite per gram of dry tissue) belonged to people affected by AD (Hautot et al., 2003). Quintana et al. (2004) used HR-STEM techniques as well as both EDXS and EELS to determine if there was a mineralogical difference in the ferritin core between samples from people who died with no known neurodegenerative disease (“healthy” tissues) and those of patients affected by neurodegenerative diseases. The authors found that ferritin retrieved from healthy tissues was composed mainly of ferrihydrite, whereas ferritin retrieved from tissues from AD and PD patients was composed mainly of magnetite and hematite (Quintana et al., 2004). Pankhurst et al. (2008) proposed that the brains of people affected by AD could synthesize biogenic magnetite at an accelerated rate. These authors studied a small sample composed of white Caucasian subjects (five male and six female patients affected by AD, with four male controls and seven female controls) and noticed that the highest magnetite concentrations and the smallest particles were all from subjects affected by AD; thus magnetite concentration could be related to the duration of the disease, age, and concomitance of other diseases. In addition, the female AD-affected group exhibited the highest magnetite concentration, suggesting a difference between sexes (Pankhurst et al., 2008). The authors of this study attributed the higher magnetite concentration in the female group to the fact that menstruation affects Fe absorption and management, and their study may have observed the long-term effects of this (Pankhurst et al., 2008). In another study, no relationship between age and magnetite concentration in females was observed in an investigation of hippocampus tissue from cases with or without epileptic seizures (but no females with AD were examined) (Dobson, 2001). The conclusions of Pankhurst et al. (2008) are not compatible with the findings of a more recent study by Hammond et al. (2021), who did not observe any significant variation in the concentration of ferrimagnetic particles between samples from AD patients and those from deceased people with no known neurodegenerative diseases. In both groups, higher concentrations of magnetite were seen in the frontal lobe in relation to the entorhinal cortex (Hammond et al., 2021). Therefore, further studies are needed in this regard, utilizing more advanced analytical techniques. Having more information about the crystallochemical nature of magnetite NPs and their localization and accumulation in the brains of both AD and healthy patients will help in determining whether indeed there may be a correlation between magnetite (biogenic or exogenous) and neurodegenerative diseases.
Plascencia-Villa et al. (2016) extracted amyloid-plaque cores from the brains of AD patients and, through HR-TEM–EDXS investigations, detected Fe NPs with spherical and elongated shapes, ranging in size from 8 to 50 nm, in direct association with the amyloid-plaque cores. These Fe NPs had inter-planar distances of 0.46 nm, which are compatible with the crystal structure of magnetite (Plascencia-Villa et al., 2016). The formation of these magnetite NPs was attributed to biological processes rather than an external pollution source (Plascencia-Villa et al., 2016). Specifically, magnetite NPs might form through the aggregation of ferrihydrite derived from ferritin or because of the presence of Aβ. The Aβ protein facilitates localized increases in redox-active Fe2+, and this process may lead to the precipitation of magnetite NPs (Everett et al., 2014a, b). More recently, Everett et al. (2021) isolated and analyzed amyloid plaques through STXM and were able to demonstrate the presence of both Fe and Cu in different chemical and redox states within the same amyloid plaque. Everett et al. (2021) hypothesized that after exposure to Fe and Cu, these elements were collected in the brain and enhanced the development of amyloid plaques. Indeed, Everett et al. (2021) were the first to report Cu0, which appeared to precipitate within amyloid-plaque cores in brain tissue. The mechanism of Cu0 precipitation in amyloid-plaque cores is still not clear. In plants, fungi, and bacteria, it has been demonstrated that Cu0 and Fe0 are enzymatic reduction products coupled with the oxidation of glucose (Jang et al., 2015; Everett et al., 2021). An overexposure of the human brain to elemental Zn and Cu may contribute to the precipitation of Aβ proteins (Bush, 2003) and, consequently, the formation of amyloid plaques.
While neurodegenerative diseases are clearly linked to an excess of certain minerals and mineraloids in the brain, there is no exhaustive explanation for the trigger mechanism(s) of these diseases (Tsolaki and Bertazzo, 2019). Even though a correlation between the valence state of Fe and neurodegenerative diseases has already been demonstrated (Kruer, 2013), the valence state is not usually determined in histochemical investigations. Most of the Fe in the body is stored in the trivalent state because Fe3+ is less reactive than Fe2+ and, unlike the bivalent form, does not produce free radicals through the Fenton reaction (Pankhurst et al., 2008). Indeed, Fe-mediated lipid peroxidation, driven by Fe2+–dioxygen chemistry or hydroxyl radical generation, is a key contributor to free-radical-induced oxidative damage in brain injury and pathology (Schafer et al., 2000). The presence of any reactive metal, like Fe or Cu, can force the brain into high oxidative stress, which may trigger neurodegenerative diseases (Bush, 2003; Kell, 2009; Everett et al., 2021).
Since PM concentration in the atmosphere is in many cases related to human activities, especially in densely populated settings, it is fundamental to consider urbanization trends, which may impact the brain mineralogy. Approximately half of the world's population now resides in urban areas, and this number is expected to grow in the coming decades (Chen et al., 2014; United Nations Department of Economic and Social Affairs, Population Division, 2019, 2022). In addition to the world's growing urban population, the number of vehicles per capita has increased as well (e.g., for the EU, Eurostat, 2023; and for the United States, EERE, 2023). In this context, the need to reduce fossil fuel emissions is driving the expansion of electric car availability (ACEA, 2023). Since electric cars are generally heavier than traditional gasoline- and diesel-burning vehicles and exhibit a higher torque, non-exhaust emissions, i.e., brake- and tire-abrasion particles, are increasing along with the number of electric cars (Fussell et al., 2022). According to a study by Querol et al. (2004), urban settings as opposed to rural and suburban sites recorded the highest concentration of crystalline phases present in PM. Some of the main contributors to the increased concentration of crystalline phases in PM include transportation-related emissions (including both exhaust and non-exhaust emissions), industrial processes, construction, and the use of fossil fuels for heating and cooking purposes (Fenger, 1999; Nyirő-Kósa et al., 2022; Power et al., 2023). Furthermore, concentrations of toxic metals in atmospheric PM are higher in urban than in rural areas (Lu et al., 2010). According to Acosta et al. (2011), who focused on the particle size distribution of atmospheric dust in Murcia (Spain), all dust particles with a diameter <2 µm derived from anthropogenic activity can adsorb larger numbers of heavy-metal ions onto their surfaces compared to “natural” dust. Indeed, other studies showed that heavy metals are more prominent in the fraction of road dust with a diameter of less than approximately <45 µm because the high specific surface area of the PM led to enhanced adsorption (Sutherland, 2003; Acosta et al., 2011; Navarro-Ciurana et al., 2023). Due to the direct relationship between specific surface area and size, UFPs are especially dangerous for humans since they can transport heavy-metal ions directly to organs, including the brain. Ferromagnetic Fe-oxides constitute the majority of the Fe-bearing particles released by vehicles (Yang et al., 2016; Nyirő-Kósa et al., 2022). These include mixtures of magnetite, maghemite, and hematite. Some metallic Fe (α-Fe) has also been recorded (Yang et al., 2016; Gonet and Maher, 2019). Crystalline Fe-(hydr)oxide phases have a high affinity to metal ions, and thus, metal ions adhere easily to Fe-(hydr)oxide surfaces (Bruemmer et al., 1988; Maher et al., 2008; Wang et al., 2017; Baragaño et al., 2021; Ledingham et al., 2024). Considering that urban PM is derived mainly from industrial activities and traffic (Zhu et al., 2013; Kaonga et al., 2021), it may act as a vector for heavy-metal pollution. To highlight this aspect, Table A2 lists research concerning cations and particles that are specifically associated with Fe-oxide minerals in different urban and industrial environments. The strong correlations and co-occurrence of Fe-oxide particles and heavy metals (both dispersed and as an identifiable solid compound) in urban spaces should be considered when identifying possible origins and exposure pathways and evaluating the toxicity of exogenous Fe-oxide minerals in the brain.
Despite considerable political efforts aimed at reducing traffic-related PM emissions, non-exhaust vehicle emissions of PM remain a substantial contributor to urban pollution (Amato et al., 2009; Hussain et al., 2014). With the steady increase in production and development of new hybrid and electric vehicles, these new technologies will undoubtedly release a variety of solid-PM-like metals and tire- and brake-abrasion particles (see e.g., Gieré and Dietze, 2022; Gieré et al., 2024). The shift in the vehicle fleet requires a better understanding of the mineralogical evolution of urban PM, with a particular focus on the mineralogical speciation in both the environment and specific organs of the human body. Due to the high population density and the concentration of many different emission sources in urban areas, atmospheric PM has become a growing concern for cities worldwide. More people living in cities leads to higher PM emissions and increased exposure rates (Zhan et al., 2023). Consequently, it is crucial to develop standard procedures for future analysis of the exogenous crystalline phases present in the brain to understand how they interact with this vital organ, in addition to other organs in the human body.
The evidence that a plethora of minerals accumulate in the human brain should be swiftly followed by in-depth research aimed at exploring the pathophysiological mechanism(s) that links the accumulation of metals, minerals, and mineraloids to their related physicochemical state and transformation and the onset of neurodegenerative diseases. The mineralogical community can contribute considerably to this topic by exploring the physicochemical state of these solids, their transformations in a healthy brain compared to that in a diseased brain, and the dynamics of growth, dissolution, and reprecipitation within specific cell and body compartments. The capacity of heavy-metal-bearing NPs to harm the nervous system is known as neurotoxicity. This damage can result in structural or functional changes, which can cause a variety of neurological diseases (Lin et al., 2023). Preventing such diseases necessitates identifying hazardous compounds, limiting human exposure, and establishing suitable safety and remediation measures (Lin et al., 2023).
An in-depth mineralogical characterization is fundamental for any detailed study of the mechanistic interaction between NPs and the biological system and to better understand and explain both the triggering mechanism(s) and the evolution of related diseases. To evaluate the possibility that any mineralogical phase may interfere with regular physiological processes and homeostasis, such as Fe homeostasis in cells, it is crucial to identify specific mineralogical phases and their physicochemical properties (Vigliaturo et al., 2018). Numerous mineralogical investigation techniques can be applied to achieve this goal. Electron microscopes (SEM-EDXS, TEM-EDXS) can be used for crystallochemical identification and morphometric research. A better resolution of crystallochemical and morphometric information is achievable with HR-S/TEM- EDXS. Furthermore, the use of HR-S/TEM-EDXS allows for the application of cutting-edge characterization techniques, which can provide additional details. For instance, dual-EELS is used to identify the valence state of heavy metals down to atomic-scale resolution (Vigliaturo et al., 2022), whereas 4D-STEM can be used to image the electric and magnetic fields of NPs (Ophus, 2019). More specifically, 4D-STEM creates an image of the magnetic field by detecting the phase gradient based on the primary beam's displacement in STEM mode as it passes through the sample and is influenced by the Lorentz force (Hachtel et al., 2018; Ophus, 2019). These latter cutting-edge methodologies represent an effective procedure for characterizing the mineralogical and physicochemical characteristics of UFPs down to the atomic scale, both ex situ and, eventually, in situ using appropriate TEM sample holders.
Because of the complexity of the nervous system and the adverse effects of neurotoxicity, it is critical to deeply understand all components, such as the minerals involved, anthropogenic phases, and biological targets, of the system studied that may contribute to the triggering and development of diseases. The evolution of the physicochemical state of solid particles and their transformation might be investigated by employing different formulations of simulated biofluids (SBFs) (Marques et al., 2011). SBFs allow for the simplification of a certain system while preserving total control over the variables of interest (such as the size, shape, and chemical composition of mineral NPs, as well as the pH, T, and pCO2 of the SBF). In vitro models (see e.g., Wohlleben et al., 2013; Oberdörster and Kuhlbusch, 2018; Vigliaturo et al., 2018; O'Shea et al., 2021c) should also be effective for studying the dissolution kinetics and ROS-formation processes of metal-bearing NPs inside the human brain. More specifically, acellular in vitro models (AIVMs, a term used when SBFs are used instead of cell cultures) represent a good complement to classic cellular in vitro investigations (Utembe et al., 2015) since AIVMs yield similar results in long-term-exposure experiments (Finch, 1990; Ansoborlo et al., 1998; Stefaniak et al., 2006; Utembe et al., 2015). During dissolution experiments, ions released through leaching may be detected using spectrometry techniques such as ICP-MS (Vigliaturo et al., 2018). Choosing AIVMs can be convenient for a multi-analytical characterization of NPs after exposure to SBFs. A preliminary study of the interaction among particles of interest and selected simulated biofluids in AIVM allows for a better interpretation of more complex systems, such as in vitro models based on cell cultures. Studies using in vitro experiments, however, are valuable for investigating cellular internalization mechanisms, particle dimensional distributions within cells (Vigliaturo et al., 2024), the structural alterations of NPs internalized by cells (Vigliaturo et al., 2022), and the biological responses to particle exposure (e.g., lung cells, Könczöl et al., 2011, 2012).
Table A1 compiles the most relevant articles related to Fe-containing minerals and mineraloids (both biogenic and exogenous) in humans and/or other animals. Table A2 lists some research that highlights the strong correlations between Fe-oxide particles and heavy metals in different urban environments, such as road dust and PM.
All the data related to this work are presented in the text of the paper (Tables A1 and A2) and in the references therein.
Conceptualization; investigation; supervision; validation; visualization; writing – original draft preparation; and writing – review and editing: GPS, CMR, RG, and RV.
At least one of the (co-)authors is a member of the editorial board of European Journal of Mineralogy. The peer-review process was guided by an independent editor, and the authors also have no other competing interests to declare.
Publisher's note: Copernicus Publications remains neutral with regard to jurisdictional claims made in the text, published maps, institutional affiliations, or any other geographical representation in this paper. While Copernicus Publications makes every effort to include appropriate place names, the final responsibility lies with the authors.
This research was supported by the Grant for Internationalization (GFI_22_01) – Mammalian Brain Mineralogy; the local research grant VIGR_RILO_23_01 1; the local research funding VIGR_RIC_N_COMP_23_0, all awarded to Ruggero Vigliaturo; and the scholarship “Borse di Studio per le Università del Piemonte, della Liguria e della Val d'Aosta Ed.2022”, Cecilia Gilardi Foundation, awarded to Giulia Pia Servetto.
This research was supported by the Grant for Internationalization (GFI_22_01) – Mammalian Brain Mineralogy; the local research grant VIGR_RILO_23_01 1; and the local research funding VIGR_RIC_N_COMP_23_0, all awarded to Ruggero Vigliaturo.
This paper was edited by Alberto Pérez-Huerta and reviewed by Mihály Pósfai and one anonymous referee.
ACEA: Interactive map – Affordability of electric cars: Correlation between market uptake and annual net income, https://www.acea.auto/figure/interactive-map-affordability-of-electric-cars-correlation-between-market-uptake-and-annual-net-income/ (last access: 7 February 2024), 12 April 2023.
Acosta, J. A., Faz, Á., Kalbitz, K., Jansen, B., and Martínez-Martínez, S.: Heavy metal concentrations in particle size fractions from street dust of Murcia (Spain) as the basis for risk assessment, J. Environ. Monitor., 13, 3087, https://doi.org/10.1039/c1em10364d 2011.
Adachi, K. and Tainosho, Y.: Characterization of heavy metal particles embedded in tire dust, Environ. Int., 30, 1009–1017, https://doi.org/10.1016/j.envint.2004.04.004, 2004.
Aizenberg, J., Hanson, J., Koetzle, T. F., Weiner, S., and Addadi, L.: Control of Macromolecule Distribution within Synthetic and Biogenic Single Calcite Crystals, J. Am. Chem. Soc., 119, 881–886, https://doi.org/10.1021/ja9628821, 1997.
Alfsen, E. M., Størmer, F. C., Njå, A., and Walløe, L.: A proposed tandem mechanism for memory storage in neurons involving magnetite and prions, Medical Hypotheses, 119, 98–101, https://doi.org/10.1016/j.mehy.2018.07.003, 2018.
Amato, F., Pandolfi, M., Viana, M., Querol, X., Alastuey, A., and Moreno, T.: Spatial and chemical patterns of PM10 in road dust deposited in urban environment, Atmos. Environ., 43, 1650–1659, https://doi.org/10.1016/j.atmosenv.2008.12.009, 2009.
Amor, M., Mathon, F. P., Monteil, C. L., Busigny, V., and Lefevre, C. T.: Iron-biomineralizing organelle in magnetotactic bacteria: function, synthesis and preservation in ancient rock samples, Environ. Microbiol., 22, 3611–3632, https://doi.org/10.1111/1462-2920.15098, 2020.
Andruşca, A.: Basal ganglia, KenHub, https://www.kenhub.com/en/library/anatomy/basal-ganglia (last access: 20 June 2024), 2023.
Ansoborlo, E., Guilminette, R. A., Hoover, M. D., Chazel, V., Houpert, P., and Henge-Napoli, M. H.: Application of in vitro dissolution tests to different uranium compounds and comparison with in vivo data, Radiat. Prot. Dosimetry, 79, 33–37, https://doi.org/10.1093/oxfordjournals.rpd.a032421, 1998.
ATSDR (AGENCY FOR TOXIC SUBSTANCES AND DISEASE REGISTRY): Toxicological Profile for Chromium, U.S. Department of Health & Human Service, Syracuse, 2000.
ATSDR: Toxicological profile for silica: US US Department of Health and Human services for toxic substances and disease registry, Sep. Chap. 2, HEALTH EFFECTS, https://www.ncbi.nlm.nih.gov/books/NBK592821/ (last access: 6 June 2024), 2019.
Baconnier, S., Lang, S. B., Polomska, M., Hilczer, B., Berkovic, G., and Meshulam, G.: Calcite microcrystals in the pineal gland of the human brain: First physical and chemical studies, Bioelectromagnetics, 23, 488–495, https://doi.org/10.1002/bem.10053, 2002.
Ballard, C., Gauthier, S., Corbett, A., Brayne, C., Aarsland, D., and Jones, E.: Alzheimer's disease, Lancet, 377, 1019–1031, https://doi.org/10.1016/s0140-6736(10)61349-9, 2011.
Banaclocha, M. A. M.: Are neuronal activity-associated magnetic fields the physical base for memory?, Medical Hypotheses, 59, 555–559, https://doi.org/10.1016/s0306-9877(02)00237-2, 2002.
Banaclocha, M. A. M., Bókkon, I., and Banaclocha, H. M.: Long-term memory in brain magnetite, Medical Hypotheses, 74, 254–257, https://doi.org/10.1016/j.mehy.2009.09.024, 2010.
Baragaño, D., Gallego, J. L. R., Menéndez-Aguado, J. M., Marina, M. A., and Sierra, C.: As sorption onto Fe-based nanoparticles and recovery from soils by means of wet high intensity magnetic separation, Chem. Eng. J., 408, 127325, https://doi.org/10.1016/j.cej.2020.127325, 2021.
Bazylinski, D. A., Lefèvre, C. T., and Schüler, D.: Magnetotactic bacteria, in: Springer eBooks, 453–494, https://doi.org/10.1007/978-3-642-30141-4_74, 2013.
Beamish, L. A., Osornio-Vargas, A. R., and Wine, E.: Air pollution: An environmental factor contributing to intestinal disease, Journal of Crohn S and Colitis, 5, 279–286, https://doi.org/10.1016/j.crohns.2011.02.017, 2011.
Beard, J. L., Connor, J. R., and Jones, B. C.: Iron in the brain, Nutrition Rev., 51, 157–170, https://doi.org/10.1111/j.1753-4887.1993.tb03096.x, 1993.
Beckwith, P. R., Ellis, J. B., Revitt, D. M., and Oldfield, F.: Heavy metal and magnetic relationships for urban source sediments, Phys. Earth Planet. In., 42, 67–75, https://doi.org/10.1016/s0031-9201(86)80009-7, 1986.
Bellinger, M. R., Wei, J., Hartmann, U., Cadiou, H., Winklhofer, M., and Banks, M. A.: Conservation of magnetite biomineralization genes in all domains of life and implications for magnetic sensing, P. Natl. Acad. Sci. USA, 119, e2108655119, https://doi.org/10.1073/pnas.2108655119, 2022.
Blakemore, R. P.: Magnetotactic bacteria, Annu. Rev. Microbiol., 36, 217–238, https://doi.org/10.1146/annurev.mi.36.100182.001245, 1982.
Boskey, A. L.: Mineralization of bones and teeth, Elements, 3, 385–391, https://doi.org/10.2113/gselements.3.6.385, 2007.
Bouras, C., Giannakopoulos, P., Good, P. F., Hsu, A., Hof, P. R., and Perl, D. P.: A laser microprobe mass analysis of trace elements in brain mineralizations and capillaries in Fahr's disease, Acta Neuropathologica, 92, 351–357, https://doi.org/10.1007/s004010050529, 1996.
Bragg, W. H.: XXX. The structure of the spinel group of crystals, The London, Edinburgh and Dublin Philosophical Magazine and Journal of Science, 30, 305–315, https://doi.org/10.1080/14786440808635400, 1915.
Brem, F., Tiefenauer, L., Fink, A., Dobson, J., and Hirt, A. M.: A mixture of ferritin and magnetite nanoparticles mimics the magnetic properties of human brain tissue, Phys. Rev. B, 73, 224427, https://doi.org/10.1103/physrevb.73.224427, 2006.
Bruemmer, G. W., Gerth, J., and Tiller, K. G.: Reaction kinetics of the adsorption and desorption of nickel, zinc and cadmium by goethite. I. Adsorption and diffusion of metals, J. Soil Sci., 39, 37–52, https://doi.org/10.1111/j.1365-2389.1988.tb01192.x, 1988.
Burghardt, T. E., Ettinger, K., Köck, B., and Hauzenberger, C.: Glass beads for road markings and other industrial usage: Crystallinity and hazardous elements, Case Studies in Construction Materials, 17, e01213, https://doi.org/10.1016/j.cscm.2022.e01213, 2022.
Bush, A. I.: Copper, zinc, and the metallobiology of Alzheimer disease, Alzheimer Disease and Associated Disorders, 17, 147–150, https://doi.org/10.1097/00002093-200307000-00005, 2003.
Bush, V. J., Moyer, T. P., Batts, K. P., and Parisi, J. E.: Essential and toxic element concentrations in fresh and formalin-fixed human autopsy tissues, Clin Chem., 41, 284–294, 1995.
Butler, R. F. and Banejree, S. K.: Theoritical single-domain size range in 35 magnetite and titanomagnetite, J. Geophys. Res., 80, 4049–4058, 1975.
Byrne, J. M., Klueglein, N., Pearce, C., Rosso, K. M., Appel, E., and Kappler, A.: Redox cycling of Fe(II) and Fe(III) in magnetite by Fe-metabolizing bacteria, Science, 347, 1473–1476, https://doi.org/10.1126/science.aaa4834, 2015.
Caballero-Gómez, H., White, H. K., O'Shea, M. J., Pepino, R., Howarth, M., and Gieré, R.: Spatial Analysis and Lead-Risk Assessment of Philadelphia, USA, Geohealth, 6, e2021GH000519, https://doi.org/10.1029/2021gh000519, 2022.
Cadiou, H. and McNaughton, P. A.: Avian magnetite-based magnetoreception: a physiologist's perspective, J. R. Soc. Interface, 7, S193–S205, https://doi.org/10.1098/rsif.2009.0423.focus, 2010.
Calderón-Garcidueñas, L., Reynoso-Robles, R., Martínez, J. V., Gómez-Maqueo-Chew, A., Pérez-Guillé, B., Mukherjee, P. S., Torres-Jardón, R., Perry, G., and Gónzalez-Maciel, A.: Prefrontal white matter pathology in air pollution exposed Mexico City young urbanites and their potential impact on neurovascular unit dysfunction and the development of Alzheimer's disease, Environ. Res., 146, 404–417, https://doi.org/10.1016/j.envres.2015.12.031, 2016.
Calderón-Garcidueñas, L., Reynoso-Robles, R., and González-Maciel, A.: Combustion and friction-derived nanoparticles and industrial-sourced nanoparticles: The culprit of Alzheimer and Parkinson's diseases, Environ. Res., 176, 108574, https://doi.org/10.1016/j.envres.2019.108574, 2019.
Calderón-Garcidueñas, L., González-Maciel, A., Reynoso-Robles, R., Hammond, J., Kulesza, R., Lachmann, I., Torres-Jardón, R., Mukherjee, P. S., and Maher, B. A.: Quadruple abnormal protein aggregates in brainstem pathology and exogenous metal-rich magnetic nanoparticles (and engineered Ti-rich nanorods). The substantia nigrae is a very early target in young urbanites and the gastrointestinal tract a key brainstem portal, Environ. Res., 191, 110139, https://doi.org/10.1016/j.envres.2020.110139, 2020.
Candy, J. M., Klinowski, J., Perry, R. H., Perry, E. K., Fairbairn, A., Oakley, A. E., Carpenter, T. A., Atack, J. R., Blessed, G., and Edwardson, J. A.: ALUMINOSILICATES AND SENILE PLAQUE FORMATION IN ALZHEIMER'S DISEASE, Lancet, 327, 354–356, https://doi.org/10.1016/s0140-6736(86)92319-6, 1986.
Casanova, M. F. and Araque, J. M.: Mineralization of the basal ganglia: implications for neuropsychiatry, pathology and neuroimaging, Psychiatry Research, 121, 59–87, https://doi.org/10.1016/s0165-1781(03)00202-6, 2003.
Castellani, R. J., Moreira, P. I., Liu, G., Dobson, J., Perry, G., Smith, M. A., and Zhu, X.: Iron: the redox-active center of oxidative stress in Alzheimer disease, Neurochem. Res., 32, 1640–1645, https://doi.org/10.1007/s11064-007-9360-7, 2007.
Chang, S. B. R. and Kirschvink, J. L.: Magnetofossils, the magnetization of sediments, and the evolution of magnetite biomineralization, Annu. Rev. Earth Planet. Sc., 17, 169–195, https://doi.org/10.1146/annurev.ea.17.050189.001125, 1989.
Chaparro, M. A. E., Posada, D. B., Chaparro, M. A. E., Molinari, D., Chiavarino, L., Alba, B., Marié, D. C., Natal, M., Böhnel, H. N., and Vaira, M.: Urban and suburban's airborne magnetic particles accumulated on Tillandsia capillaris, Sci. Total Environ., 907, 167890, https://doi.org/10.1016/j.scitotenv.2023.167890, 2024.
Chasteen, N. D. and Harrison, P. M.: Mineralization in Ferritin: an efficient means of iron storage, J. Struct. Biol., 126, 182–194, https://doi.org/10.1006/jsbi.1999.4118, 1999.
Chen, M., Zhang, H., Liu, W., and Zhang, W.: The Global Pattern of Urbanization and Economic Growth: Evidence from the Last Three Decades, PloS One, 9, e103799, https://doi.org/10.1371/journal.pone.0103799, 2014.
Cheng, K. H., Cheng, Y. S., Yeh, H. C., Guilmette, R. A., Simpson, S. Q., Yang, S. Q., and Swift, D. L.: In Vivo Measurements of Nasal Airway Dimensions and Ultrafine Aerosol Depositing in Human Nasal and Oral Airways, J. Aerosol Sci., 27, 785–801, 1993.
Christiansen, M. G., Senko, A. W., and Anikeeva, P.: Magnetic Strategies for Nervous system Control, Annu. Rev. Neurosci., 42, 271–293, https://doi.org/10.1146/annurev-neuro-070918-050241, 2019.
Clark, D. L., Boutros, N. N., and Mendez, M. F.: The brain and behavior, https://doi.org/10.1017/cbo9780511776915, 2010.
Collingwood, J. F., Chong, R. K. K., Kasama, T., Cervera-Gontard, L., Dunin-Borkowski, R. E., Perry, G., Pósfai, M., Siedlak, S. L., Simpson, E. T., Smith, M. A., and Dobson, J.: Three-Dimensional Tomographic Imaging and Characterization of Iron Compounds within Alzheimer's Plaque Core Material, Journal of Alzheimer's Disease, 14, 235–245, https://doi.org/10.3233/jad-2008-14211, 2008.
Connor, J. R., Menzies, S. L., Burdo, J. R., and Boyer, P. J.: Iron and iron management proteins in neurobiology, Pediatric Neurology, 25, 118–129, https://doi.org/10.1016/s0887-8994(01)00303-4, 2001.
Connor-Stroud, F. R., Hopkins, W. D., Preuss, T. M., Johnson, Z., Zhang, X., and Sharma, P.: Extensive Vascular Mineralization in the Brain of a Chimpanzee (Pan troglodytes), Comparative Medicine, American Association for Laboratory Animal Science, PMCID: PMC4067587, 2014.
Correale, J. and Villa, A.: Cellular elements of the Blood-Brain barrier, Neurochem. Res., 34, 2067–2077, https://doi.org/10.1007/s11064-009-0081-y, 2009.
Coste, B., Mathur, J., Schmidt, M., Earley, T. J., Ranade, S., Petrus, M. J., Dubin, A. E., and Patapoutian, A.: PIEzo1 and PIEzo2 are essential components of distinct mechanically activated cation channels, Science, 330, 55–60, https://doi.org/10.1126/science.1193270, 2010.
Cumings, J. N.: THE COPPER AND IRON CONTENT OF BRAIN AND LIVER IN THE NORMAL AND IN HEPATO-LENTICULAR DEGENERATION, Brain, 71, 410–415, https://doi.org/10.1093/brain/71.4.410, 1948.
De Brouwere, K., Buekers, J., Cornelis, C., Schlekat, C. E., and Oller, A. R.: Assessment of indirect human exposure to environmental sources of nickel: Oral exposure and risk characterization for systemic effects, Sci. Total Environ., 419, 25–36, https://doi.org/10.1016/j.scitotenv.2011.12.049, 2012.
Delmas, P., Hao, J., and Rodat-Despoix, L.: Molecular mechanisms of mechanotransduction in mammalian sensory neurons, Nat. Rev., Neuroscience, 12, 139–153, https://doi.org/10.1038/nrn2993, 2011.
Diaz-Ricci, J. C. and Kirschvink, J. L.: Magnetic domain state and coercivity predictions for biogenic greigite (Fe3S4): a comparison of theory with magnetosome observations, J. Geophys. Res., 97, 17309–17315, 1992.
Dietrich, M., Huling, J., and Krekeler, M. P. S.: Metal pollution investigation of Goldman Park, Middletown Ohio: Evidence for steel and coal pollution in a high child use setting, Sci. Total Environ., 618, 1350–1362, https://doi.org/10.1016/j.scitotenv.2017.09.246, 2018.
Dietrich, M., Krekeler, M. P. S., Kousehlar, M., and Widom, E.: Quantification of Pb pollution sources in complex urban environments through a multi-source isotope mixing model based on Pb isotopes in lichens and road sediment, Environ. Pollut., 288, 117815, https://doi.org/10.1016/j.envpol.2021.117815, 2021.
Dietrich, M., O'Shea, M. J., Gieré, R., and Krekeler, M. P. S.: Road sediment, an underutilized material in environmental science research: A review of perspectives on United States studies with international context, J. Hazard. Mater., 432, 128604, https://doi.org/10.1016/j.jhazmat.2022.128604, 2022.
Dobson, J.: Nanoscale biogenic iron oxides and neurodegenerative disease, FEBS Lett., 496, 1–5, https://doi.org/10.1016/s0014-5793(01)02386-9, 2001.
Dobson, J. and Grassi, P.: Magnetic properties of human hippocampal tissue – Evaluation of artefact and contamination sources, Brain Res. Bull., 39, 255–259, https://doi.org/10.1016/0361-9230(95)02132-9, 1996.
Donaldson, K., Stone, V., Seaton, A., and MacNee, W.: Ambient particle inhalation and the cardiovascular system: potential mechanisms, Environ. Health Persp., 109, 523–527, https://doi.org/10.1289/ehp.01109s4523, 2001.
Dotiwala, A. K., McCausland, C., and Samra, N. S.: Anatomy, head and neck: blood brain barrier, StatPearls – NCBI Bookshelf, https://www.ncbi.nlm.nih.gov/books/NBK519556/ (last access: 16 April 2025), 2023.
Duer, M. J., Friščić, T., Murray, R. C., Reid, D. G., and Wise, E. R.: The Mineral Phase of Calcified Cartilage: Its Molecular Structure and Interface with the Organic Matrix, Biophys. J., 96, 3372–3378, https://doi.org/10.1016/j.bpj.2008.12.3954, 2009.
Duflou, H., Maenhaut, W., and De Reuck, J.: Regional distribution of potassium, calcium, and six trace elements in normal human brain, Neurochem. Res., 14, 1099–1112, https://doi.org/10.1007/bf00965616, 1989.
Edelman, N. B., Fritz, T., Nimpf, S., Pichler, P., Lauwers, M., Hickman, R. W., Papadaki-Anastasopoulou, A., Ushakova, L., Heuser, T., Resch, G. P., Saunders, M., Shaw, J. A., and Keays, D. A.: No evidence for intracellular magnetite in putative vertebrate magnetoreceptors identified by magnetic screening, P. Natl. Acad. Sci. USA, 112, 262–267, https://doi.org/10.1073/pnas.1407915112, 2014.
EERE: FOTW #1305, 28 August 2023: Growth in the Number of Vehicles in the U.S. Outpaced Growth in Population and Licensed Drivers from 1960 to 2021, https://www.energy.gov/eere/vehicles/articles/fotw-1305-august-28-2023-growth-number-vehicles-us-outpaced-growth (last access: 6 June 2024), 2023.
Eggleton, R. A. and Fitzpatrick, R. W.: New data and a revised structural model for ferrihydrite, Clays Clay Miner., 36, 111–124, https://doi.org/10.1346/ccmn.1988.0360203, 1988.
EPA: IRIS Toxicological Review of Chromium VI (1998 Final), U.S. Environmental Protection Agency, Washington, DC, 1998.
European Commission, EC: Opinion of the scientific committee on food on the risks to human health of polycyclic aromatic hydrocarbons in food, SCF/CS/CNTM/PAH/29 Final, 4 December 2002.
Eurostat: Database – Transport, 2023, https://ec.europa.eu/eurostat/en/web/products-eurostat-news/w/ddn-20230530-1 (last access: 20 February 2025), 2023.
Everett, J., Céspedes, E., Shelford, L. R., Exley, C., Collingwood, J. F., Dobson, J., Van Der Laan, G., Jenkins, C. A., Arenholz, E., and Telling, N. D.: Evidence of Redox-Active iron formation following aggregation of ferrihydrite and the Alzheimer's disease peptide β-Amyloid, Inorganic Chemistry, 53, 2803–2809, https://doi.org/10.1021/ic402406g, 2014a.
Everett, J., Céspedes, E., Shelford, L. R., Exley, C., Collingwood, J. F., Dobson, J., Van Der Laan, G., Jenkins, C. A., Arenholz, E., and Telling, N. D.: Ferrous iron formation following the co-aggregation of ferric iron and the Alzheimer's disease peptide β-amyloid (1–42), J. R. Soc. Interface, 11, 20140165, https://doi.org/10.1098/rsif.2014.0165, 2014b.
Everett, J., Collingwood, J. F., Tjendana-Tjhin, V., Brooks, J., Lermyte, F., Plascencia-Villa, G., Hands-Portman, I., Dobson, J., Perry, G., and Telling, N. D.: Nanoscale synchrotron X-ray speciation of iron and calcium compounds in amyloid plaque cores from Alzheimer's disease subjects, Nanoscale, 10, 11782–11796, https://doi.org/10.1039/c7nr06794a, 2018.
Everett, J., Lermyte, F., Brooks, J., Tjendana-Tjhin, V., Plascencia-Villa, G., Hands-Portman, I., Donnelly, J. M., Billimoria, K., Perry, G., Zhu, X., Sadler, P. J., O'Connor, P. B., Collingwood, J. F., and Telling, N. D.: Biogenic metallic elements in the human brain?, Sci. Adv., 7, https://doi.org/10.1126/sciadv.abf6707, 2021.
Exley, C. and House, E. R.: Aluminium in the human brain, Monatshefte Für Chemie, 142, 357–363, https://doi.org/10.1007/s00706-010-0417-y, 2010.
Exley, C. and Mold, M. J.: Imaging of aluminium and amyloid β in neurodegenerative disease, Heliyon, 6, e03839, https://doi.org/10.1016/j.heliyon.2020.e03839, 2020.
Exley, C., Mamutse, G., Korchazhkina, O., Pye, E., Strekopytov, S., Polwart, A., and Hawkins, C.: Elevated urinary excretion of aluminium and iron in multiple sclerosis, Multiple Sclerosis, 12, 533–540, https://doi.org/10.1177/1352458506071323, 2006.
Faivre, D. and Schüler, D.: Magnetotactic bacteria and magnetosomes, Chem. Rev., 108, 4875–4898, https://doi.org/10.1021/cr078258w, 2008.
Fang, W., Yang, Y., and Xu, Z.: PM10 and PM2.5 and health risk assessment for heavy metals in a typical factory for Cathode Ray tube television recycling, Environ. Sci. Technol., 47, 12469–12476, https://doi.org/10.1021/es4026613, 2013.
Farooqui, A. A.: Neurochemical aspects of neurological disorders, Elsevier eBooks, 237–256, https://doi.org/10.1016/B978-0-12-803603-7.00016-1, 2016.
Fatima, R., Akhtar, K., Hossain, M. M., and Ahmad, R.: Chromium oxide nanoparticle–induced biochemical and histopathological alterations in the kidneys and brain of Wistar rats, Toxicology and Industrial Health, 33, 911–921, https://doi.org/10.1177/0748233717735266, 2017.
Feigin, V. L., Nichols, E., Alam, T., et al.: Global, regional, and national burden of neurological disorders, 1990–2016: a systematic analysis for the Global Burden of Disease Study 2016, Lancet Neurology, 18, 459–480, https://doi.org/10.1016/s1474-4422(18)30499-x, 2019.
Fenger, J.: Urban air quality, Atmos. Environ., 33, 4877–4900, https://doi.org/10.1016/s1352-2310(99)00290-3, 1999.
Finch, G.: Clearance, translocation, and excretion of beryllium following acute inhalation of beryllium oxide by beagle dogs*1, Fund. Appl. Toxicol., 15, 231–241, https://doi.org/10.1016/0272-0590(90)90050-t, 1990.
Firlar, E., Perez-Gonzalez, T., Olszewska, A., Faivre, D., and Prozorov, T.: Following iron speciation in the early stages of magnetite magnetosome biomineralization, Journal of Materials Research/Pratt's Guide to Venture Capital Sources, 31, 547–555, https://doi.org/10.1557/jmr.2016.33, 2016.
Fischl, B. and Dale, A. M.: Measuring the thickness of the human cerebral cortex from magnetic resonance images, P. Natl. Acad. Sci. USA, 97, 11050–11055, https://doi.org/10.1073/pnas.200033797, 2000.
Fleet, M. E.: The structure of magnetite, Acta Crystallogr. B, 37, 917–920, https://doi.org/10.1107/s0567740881004597, 1981.
Francis, E. A., Xiao, H., Teng, L. H., and Heinrich, V.: Mechanisms of frustrated phagocytic spreading of human neutrophils on antibody-coated surfaces, Biophys. J., 121, 4714–4728, https://doi.org/10.1016/j.bpj.2022.10.016, 2022.
Frankel, R. B., Blakemore, R. P., and Wolfe, R. S.: Magnetite in freshwater magnetotactic bacteria, Science, 203, 1355–1356, https://doi.org/10.1126/science.203.4387.1355, 1979.
Freundlich, M.: Infant formula as a cause of aluminium toxicity in neonatal uraemiA, Lancet, 326, 527–529, https://doi.org/10.1016/s0140-6736(85)90463-5, 1985.
Friedman, J. H. and Chou, K. L.: Mood, Emotion, and Thought, Textbook of Clinical Neurology, edited by: Goetz, C. G., 3rd edn., Chap. 3, ISBN 978-1-4160-3618-0, 2007.
Friedman, A., Arosio, P., Finazzi, D., Koziorowski, D., and Galazka-Friedman, J.: Ferritin as an important player in neurodegeneration, Parkinsonism & Related Disorders (Online)/Parkinsonism & Related Disorders, 17, 423–430, https://doi.org/10.1016/j.parkreldis.2011.03.016, 2011.
Fukuda, Y., Okamura, Y., Takeyama, H., and Matsunaga, T.: Dynamic analysis of a genomic island in Magnetospirillum sp. strain AMB-1 reveals how magnetosome synthesis developed, FEBS Lett., 580, 801–812, https://doi.org/10.1016/j.febslet.2006.01.003, 2006.
Fussell, J. C., Franklin, M., Green, D. C., Gustafsson, M., Harrison, R. M., Hicks, W., Kelly, F. J., Kishta, F., Miller, M. R., Mudway, I. S., Oroumiyeh, F., Selley, L., Wang, M., and Zhu, Y.: A review of Road Traffic-Derived Non-Exhaust Particles: emissions, physicochemical characteristics, health risks, and mitigation measures, Environ. Sci. Technol., 56, 6813–6835, https://doi.org/10.1021/acs.est.2c01072, 2022.
Gálvez, N., Fernández, B., Sánchez, P., Cuesta, R., Ceolín, M., Clemente-León, M., Trasobares, S., López-Haro, M., Calvino, J. J., Stéphan, O., and Domínguez-Vera, J. M.: Comparative Structural and Chemical Studies of Ferritin Cores with Gradual Removal of their Iron Contents, J. Am. Chem. Soc., 130, 8062–8068, https://doi.org/10.1021/ja800492z, 2008.
Gautam, P., Blaha, U., and Appel, E.: Magnetic susceptibility of dust-loaded leaves as a proxy of traffic-related heavy metal pollution in Kathmandu city, Nepal, Atmos. Environ., 39, 2201–2211, https://doi.org/10.1016/j.atmosenv.2005.01.006, 2005.
Gawdi, R., Shumway, K. R., and Emmady, P. D.: Physiology, Blood Brain Barrier, in: StatPearls, Treasure Island (FL), StatPearls, PMID: 32491653, 2023.
Gellerstedt, N.: Our knowledge of cerebral changes in normal involution of old age, Uppsala Läk.-Fören, Förh., 38, 194–408, 1933.
Gieré, R. and Dietze, V.: Tire-Abrasion particles in the environment, Adv. Polymer Sci., 71–101, https://doi.org/10.1007/12_2022_118, 2022.
Gieré, R. and Querol, X.: Solid particulate matter in the atmosphere, Elements, 6, 215–222, https://doi.org/10.2113/gselements.6.4.215, 2010.
Gieré, R., Carleton, L. E., and Lumpkin, G. R.: Micro- and nanochemistry of fly ash from a coal-fired power plant, Am. Mineral., 88, 1853–1865, https://doi.org/10.2138/am-2003-11-1228, 2003.
Gieré, R., Edwards, J., Dietze, V., Stoček, R., and Heinrich, G: Vehicle tyre particles in the environment – Foresight Brief No. 034, August 2024, https://doi.org/10.59117/20.500.11822/46239, 2024.
Gilles, C., Bonville, P., Rakoto, H., Broto, J. M., Wong, K. K. W., and Mann, S.: Magnetic hysteresis and superantiferromagnetism in ferritin nanoparticles, J. Magn. Magn. Mater., 241, 430–440, https://doi.org/10.1016/s0304-8853(01)00461-9, 2002.
Gonet, T. and Maher, B. A.: Airborne, Vehicle-Derived Fe-Bearing Nanoparticles in the Urban Environment: A review, Environ. Sci. Technol., 53, 9970–9991, https://doi.org/10.1021/acs.est.9b01505, 2019.
Goodman, L.: Alzheimer's disease, J. Nerv. Ment. Dis., 118, 97–130, https://doi.org/10.1097/00005053-195308000-00001, 1953.
Gorby, Y. A., Beveridge, T. J., and Blakemore, R. P.: Characterization of the bacterial magnetosome membrane, J. Bacteriol., 170, 834–841, https://doi.org/10.1128/jb.170.2.834-841.1988, 1988.
Gorell, J. M., Johnson, C. C., Rybicki, B. A., Peterson, E. L., Kortsha, G. X., Brown, G. G., and Richardson, R. J.: Occupational exposures to metals as risk factors for Parkinson's disease, Neurology, 48, 650–658, https://doi.org/10.1212/wnl.48.3.650, 1997.
Gorobets, O., Gorobets, S., and Koralewski, M.: Physiological origin of biogenic magnetic nanoparticles in health and disease: from bacteria to humans, Int. J. Nanomed., 12, 4371–4395, https://doi.org/10.2147/ijn.s130565, 2017.
Gorobets, O. Yu., Gorobets, S. V., and Gorobets, Yu. I.: Biogenic Magnetic Nanoparticles: Biomineralization in Prokaryotes and Eukaryotes, Dekker Encyclopedia of Nanoscience and Nanotechnology, 300–308, ISBN 9781439891346, 2014.
Gorobets, S. V., Yu, G. O., Demianenko, I. V., and Nikolaenko, R. N.: Self-organization of magnetite nanoparticles in providing Saccharomyces cerevisiae Yeasts with magnetic properties, J. Magn. Magn. Mater., 337–338, 53–57, https://doi.org/10.1016/j.jmmm.2013.01.004, 2013.
Gould, J. L., Kirschvink, J. L., and Deffeyes, K. S.: Bees have magnetic remanence, Science, 201, 1026–1028, https://doi.org/10.1126/science.201.4360.1026, 1978.
Goychuk, I.: Sensing Magnetic Fields with Magnetosensitive Ion Channels, Sensors, 18, 728, https://doi.org/10.3390/s18030728, 2018.
Gray, H. and Lewis, W. H.: Anatomy of the Human Body, Philadelphia and New York, Lea & Febiger, Retrieved from the Library of Congress, https://lccn.loc.gov/18017427 (last access: 22 April 2025), 1918.
Grobéty, B., Giere, R., Dietze, V., and Stille, P.: Airborne particles in the urban environment, Elements, 6, 229–234, https://doi.org/10.2113/gselements.6.4.229, 2010.
Hachtel, J. A., Idrobo, J. C., and Chi, M.: Sub-Ångstrom electric field measurements on a universal detector in a scanning transmission electron microscope, Adv. Struct. Chem. Imag., 4, 10, https://doi.org/10.1186/s40679-018-0059-4, 2018.
Hadfield, G.: Siderosis of the globus pallidus: Its relation to bilateral necrosis, J. Pathol., 32, 135–148, https://doi.org/10.1002/path.1700320116, 1929.
Hallgren, B. and Sourander, P.: the effect of age on the non-haemin iron in the human brain, J. Neurochem., 3, 41–51, https://doi.org/10.1111/j.1471-4159.1958.tb12607.x, 1958.
Halsall, C. J., Maher, B. A., Karloukovski, V. V., Shah, P., and Watkins, S. J.: A novel approach to investigating indoor/outdoor pollution links: Combined magnetic and PAH measurements, Atmos. Environ., 42, 8902–8909, https://doi.org/10.1016/j.atmosenv.2008.09.001, 2008.
Hammond, J., Maher, B. A., Ahmed, I. a. M., and Allsop, D.: Variation in the concentration and regional distribution of magnetic nanoparticles in human brains, with and without Alzheimer's disease, from the UK, Sci. Rep., 11, 9363, https://doi.org/10.1038/s41598-021-88725-3, 2021.
Hanzlik, M., Heunemann, C., Holtkamp-Rötzler, E., Winklhofer, M., Petersen, N., and Fleissner, G.: Superparamagnetic magnetite in the upper beak tissue of homing pigeons, BioMetals, 13, 325–331, https://doi.org/10.1023/a:1009214526685, 2000.
Harrison, P. M. and Arosio, P.: The ferritins: molecular properties, iron storage function and cellular regulation, Biochimica Et Biophysica Acta, Bioenergetics, 1275, 161–203, https://doi.org/10.1016/0005-2728(96)00022-9, 1996.
Hautot, D., Pankhurst, Q. A., Khan, N., and Dobson, J.: Preliminary evaluation of nanoscale biogenic magnetite in Alzheimer's disease brain tissue, Proceedings – Royal Society. Biological Sciences/Proceedings – Royal Society. Biological Sciences, 270, S62–S64, https://doi.org/10.1098/rsbl.2003.0012, 2003.
Honarpisheh, M., Foresto-Neto, O., Desai, J., Steiger, S., Gómez, L. A., Popper, B., Boor, P., Anders, H.-J., and Mulay, S. R.: Phagocytosis of environmental or metabolic crystalline particles induces cytotoxicity by triggering necroptosis across a broad range of particle size and shape, Sci. Rep., 7, 15523, https://doi.org/10.1038/s41598-017-15804-9, 2017.
Huber, D. L.: Synthesis, properties, and applications of iron nanoparticles, Small, 1, 482–501, https://doi.org/10.1002/smll.200500006, 2005.
Hughes, S., McBain, S., Dobson, J., and Haj, A. J. E.: Selective activation of mechanosensitive ion channels using magnetic particles, J. R. Soc. Interface, 5, 855–863, https://doi.org/10.1098/rsif.2007.1274, 2007.
Hunter, D. D. and Dey, R. D.: Identification and neuropeptide content of trigeminal neurons innervating the rat nasal epithelium, Neuroscience, 83, 591–599, https://doi.org/10.1016/s0306-4522(97)00324-2, 1998.
Hunter, D. D. and Undem, B. J.: Identification and substance P content of vagal afferent neurons innervating the epithelium of the guinea pig trachea, Am. J. Resp. Crit. Care, 159, 1943–1948, https://doi.org/10.1164/ajrccm.159.6.9808078, 1999.
Hussain, S., Hamid, M. K. A., Lazim, A. R. M., and Bakar, A. R. A.: Brake Wear Particle size and shape analysis of Non-Asbestos Organic (NAO) and Semi Metallic brake pad, Jurnal Teknologi/Jurnal Teknologi, 71, https://doi.org/10.11113/jt.v71.3731, 2014.
Jaishankar, M., Tseten, T., Anbalagan, N., Mathew, B. B., and Beeregowda, K. N.: Toxicity, mechanism and health effects of some heavy metals, Interdisciplinary Toxicology, 7, 60–72, https://doi.org/10.2478/intox-2014-0009, 2014.
Jang, G. G., Jacobs, C. B., Gresback, R. G., Ivanov, I. N., Meyer, H. M., Kidder, M., Joshi, P. C., Jellison, G. E., Phelps, T. J., Graham, D. E., and Moon, J. W.: Size tunable elemental copper nanoparticles: extracellular synthesis by thermoanaerobic bacteria and capping molecules, J. Mater. Chem. C, 3, 644–650, https://doi.org/10.1039/c4tc02356k, 2015.
Järup, L.: Hazards of heavy metal contamination, Brit. Med. Bull., 68, 167–182, https://doi.org/10.1093/bmb/ldg032, 2003.
Jones, L. K.: Anatomy and Brain Development, American Counseling Association, 1–26, https://doi.org/10.1002/9781119375487.ch1, 2017.
Kampa, M. and Castanas, E.: Human health effects of air pollution, Environ. Pollut., 151, 362–367, https://doi.org/10.1016/j.envpol.2007.06.012, 2007.
Kanapilly, G. M., Wolff, R. K., DeNee, P. B., and McClellan, R. O.: Generation, characterization and inhalation deposition of ultrafine aggregate aerosols, Ann. Occup. Hyg., 26, 77–91, 1982.
Kaonga, C. C., Kosamu, I. B. M., and Utembe, W. R.: A review of metal levels in urban dust, their methods of determination, and risk assessment, Atmosphere, 12, 891, https://doi.org/10.3390/atmos12070891, 2021.
Kelepertzis, E., Argyraki, A., Botsou, F., Aidona, E., Szabó, Á., and Szabó, C.: Tracking the occurrence of anthropogenic magnetic particles and potentially toxic elements (PTEs) in house dust using magnetic and geochemical analyses, Environ. Pollut., 245, 909–920, https://doi.org/10.1016/j.envpol.2018.11.072, 2019.
Kell, D. B.: Iron behaving badly: inappropriate iron chelation as a major contributor to the aetiology of vascular and other progressive inflammatory and degenerative diseases, BMC Medical Genomics, 2, 2, https://doi.org/10.1186/1755-8794-2-2, 2009.
Kirschvink, J. L.: Comment on “Constraints on biological effects of weak extremely-low-frequency electromagnetic fields”, Physical Review. A, Atomic, Molecular, and Optical Physics/Physical Review, a, Atomic, Molecular, and Optical Physics, 46, 2178–2184, https://doi.org/10.1103/physreva.46.2178, 1992.
Kirschvink, J. L. and Gould, J. L.: Biogenic magnetite as a basis for magnetic field detection in animals, Biosystems, 13, 181–201, https://doi.org/10.1016/0303-2647(81)90060-5, 1981.
Kirschvink, J. L. and Hagadorn, J. W.: A Grand Unified Theory of Biomineralization, in: Biomineralization. Progress in Biology, Molecular Biology and Application, edited by: Bäuerlein, E., 139–150, Wiley-VCH Verlag, Weinheim, Germany, 2000.
Kirschvink, J. L., Jones, D. S., and MacFadden, B. J.: Magnetite biomineralization and magnetoreception in organisms, Springer Nature Link, https://doi.org/10.1007/978-1-4613-0313-8, 1985.
Kirschvink, J. L., Kobayashi-Kirschvink, A., and Woodford, B. J.: Magnetite biomineralization in the human brain, P. Natl. Acad. Sci. USA, 89, 7683–7687, https://doi.org/10.1073/pnas.89.16.7683, 1992.
Könczöl, M., Ebeling, S., Goldenberg, E., Treude, F., Gminski, R., Gieré, R., Grobéty, B., Rothen-Rutishauser, B., Merfort, I., and Mersch-Sundermann, V.: Cytotoxicity and genotoxicity of Size-Fractionated iron oxide (Magnetite) in a549 human lung epithelial cells: role of ROS, JNK, and NF-KB, Chem. Res. Toxicol., 24, 1460–1475, https://doi.org/10.1021/tx200051s, 2011.
Könczöl, M., Goldenberg, E., Ebeling, S., Schäfer, B., Garcia-Käufer, M., Gminski, R., Grobéty, B., Rothen-Rutishauser, B., Merfort, I., Gieré, R., and Mersch-Sundermann, V.: Cellular uptake and toxic effects of fine and ultrafine Metal-Sulfate particles in human A549 lung epithelial cells, Chem. Res. Toxicol., 25, 2687–2703, https://doi.org/10.1021/tx300333z, 2012.
Kozlenko, D. P., Dubrovinsky, L., Kichanov, S. E., Lukin, E. V., Cerantola, V., Chumakov, A. I., and Savenko, B. N.: Magnetic and electronic properties of magnetite across the high pressure anomaly, Sci. Rep., 9, 4464, https://doi.org/10.1038/s41598-019-41184-3, 2019.
Kreuter, J., Shamenkov, D., Petrov, V., Ramge, P., Cychutek, K., Koch-Brandt, C., and Alyautdin, R.: Apolipoprotein-mediated transport of nanoparticle-bound drugs across the Blood-Brain barrier, J. Drug Targeting, 10, 317–325, https://doi.org/10.1080/10611860290031877, 2002.
Kreutzer, J. S., DeLuca, J., and Caplan, B.: Encyclopedia of Clinical Neuropsychology, https://doi.org/10.1007/978-0-387-79948-3, 2011.
Kreyling, W. G., Blanchard, J. D., Godleski, J. J., Haeussermann, S., Heyder, J., Hutzler, P., Schulz, H., Sweeney, T. D., Takenaka, S., and Ziesenis, A.: Anatomic localization of 24- and 96-h particle retention in canine airways, J. Appl. Physiol., 87, 269–284, https://doi.org/10.1152/jappl.1999.87.1.269, 1999.
Krichen, S., Liu, L., and Sharma, P.: Biological cell as a soft magnetoelectric material: Elucidating the physical mechanisms underpinning the detection of magnetic fields by animals, Phys. Rev. E, 96, 042404, https://doi.org/10.1103/physreve.96.042404, 2017.
Kruer, M. C.: The Neuropathology of Neurodegeneration with Brain Iron Accumulation, in: International review of neurobiology, 165–194, https://doi.org/10.1016/b978-0-12-410502-7.00009-0, 2013.
Kwon, H.-S., Ryu, M. H., and Carlsten, C.: Ultrafine particles: unique physicochemical properties relevant to health and disease, Exp. Molec. Med., 52, 318–328, https://doi.org/10.1038/s12276-020-0405-1, 2020.
Labarta, A., Batlle, X., and Iglesias, Ò.: From finite size and surface effects to glassy behaviour in ferrimagnetic nanoparticles, in: Springer eBooks, 105–140, https://doi.org/10.1007/0-387-26018-8_4, 2005.
Lai, C.-Y., Lai, C.-H., Chuang, H.-C., Pan, C.-H., Yen, C.-C., Lin, W.-Y., Chen, J.-K., Lin, L.-Y., and Chuang, K.-J.: Physicochemistry and cardiovascular toxicity of metal fume PM2.5: a study of human coronary artery endothelial cells and welding workers, Sci. Rep., 6, 33515, https://doi.org/10.1038/srep33515, 2016.
Landsberg, J. P., McDonald, B., and Watt, F.: Absence of aluminium in neuritic plaque cores in Alzheimer's disease, Nature, 360, 65–68, https://doi.org/10.1038/360065a0, 1992.
Ledingham, G. J., Fang, Y., and Catalano, J. G.: Irreversible trace metal binding to goethite controlled by the ion size, Environ. Sci. Technol., 58, 2007–2016, https://doi.org/10.1021/acs.est.3c06516, 2024.
Lee, J.-H., Kim, J.-W., Levy, M., Kao, A., Noh, S.-H., Bozovic, D., and Cheon, J.: Magnetic nanoparticles for ultrafast mechanical control of inner ear hair cells, ACS Nano, 8, 6590–6598, https://doi.org/10.1021/nn5020616, 2014.
Leikauf, G. D., Kim, S. H., and Jang, A. S.: Mechanisms of ultrafine particle-induced respiratory health effects, Exp. Molec. Med., 52, 329–337, https://doi.org/10.1038/s12276-020-0394-0, 2020.
Lewinson, J., Mayr, W., and Wagner, H.: Characterization and toxicological behavior of synthetic amorphous hydrophobic silica, Regulatory Toxicology and Pharmacology, 20, 37–57, https://doi.org/10.1006/rtph.1994.1035, 1994.
Li, N., Georas, S., Alexis, N., Fritz, P., Xia, T., Williams, M. A., Horner, E., and Nel, A.: A work group report on ultrafine particles (American Academy of Allergy, Asthma & Immunology): Why ambient ultrafine and engineered nanoparticles should receive special attention for possible adverse health outcomes in human subjects, J. Allergy Clin. Immun., 138, 386–396, https://doi.org/10.1016/j.jaci.2016.02.023, 2016.
Li, Q., Kartikowati, C. W., Horie, S., Ogi, T., Iwaki, T., and Okuyama, K.: Correlation between particle size/domain structure and magnetic properties of highly crystalline Fe3O4 nanoparticles, Sci. Rep., 7, 9894, https://doi.org/10.1038/s41598-017-09897-5, 2017.
Lin, W., Huang, Z., Zhang, W., and Ren, Y.: Investigating the neurotoxicity of environmental pollutants using zebrafish as a model organism: A review and recommendations for future work, Neurotoxicology, 94, 235–244, https://doi.org/10.1016/j.neuro.2022.12.009, 2023.
Loeb, J. A., Sohrab, S. A., Huq, M., and Fuerst, D. R.: Brain calcifications induce neurodegenerative dysfunction that can be reversed by a bone drug, Journal of the Neurodegenerative Sciences, 243, 77–81, https://doi.org/10.1016/j.jns.2005.11.033, 2006.
Lohße, A., Ullrich, S., Katzmann, E., Borg, S., Wanner, G., Richter, M., Voigt, B., Schweder, T., and Schüler, D.: Functional Analysis of the Magnetosome Island in Magnetospirillum gryphiswaldense: The mamAB Operon Is Sufficient for Magnetite Biomineralization, PloS One, 6, e25561, https://doi.org/10.1371/journal.pone.0025561, 2011.
Lomer, M. C. E., Thompson, R. P. H., and Powell, J. J.: Fine and ultrafine particles of the diet: influence on the mucosal immune response and association with Crohn's disease, P. Nutr. Soc., 61, 123–130, https://doi.org/10.1079/pns2001134, 2002.
Lomer, M. C. E., Hutchinson, C., Volkert, S., Greenfield, S. M., Catterall, A., Thompson, R. P. H., and Powell, J. J.: Dietary sources of inorganic microparticles and their intake in healthy subjects and patients with Crohn's disease, Brit. J. Nutr., 92, 947–955, https://doi.org/10.1079/bjn20041276, 2004.
Lovell, M. A., Robertson, J. D., Teesdale, W. J., Campbell, J. L., and Markesbery, W. R.: Copper, iron and zinc in Alzheimer's disease senile plaques, J. Neurolog. Sci., 158, 47–52, https://doi.org/10.1016/s0022-510x(98)00092-6, 1998.
Lowenstam, H. A.: Magnetite in Denticle Capping in Recent Chitons (Polyplacophora), GSA Bull., 73, 435–438, https://doi.org/10.1130/0016-7606(1962)73[435:MIDCIR]2.0.CO;2, 1962.
Lu, X., Wang, L., Li, L. Y., Lei, K., Huang, L., and Kang, D.: Multivariate statistical analysis of heavy metals in street dust of Baoji, NW China, J. Hazard. Mater., 173, 744–749, https://doi.org/10.1016/j.jhazmat.2009.09.001, 2010.
Maetzler, W., Berg, D., Funke, C., Sandmann, F., Stünitz, H., Maetzler, C., and Nitsch, C.: Progressive secondary neurodegeneration and microcalcification Co-OCCuR in Osteopontin-Deficient mice, Am. J. Pathol., 177, 829–839, https://doi.org/10.2353/ajpath.2010.090798, 2010.
Maher, B. A.: Airborne magnetite- and Iron-Rich pollution nanoparticles: potential neurotoxicants and environmental risk factors for neurodegenerative disease, including Alzheimer's disease, Journal of Alzheimer's Disease, 71, 361–375, https://doi.org/10.3233/jad-190204, 2019.
Maher, B. A., Moore, C., and Matzka, J.: Spatial variation in vehicle-derived metal pollution identified by magnetic and elemental analysis of roadside tree leaves, Atmos. Environ., 42, 364–373, https://doi.org/10.1016/j.atmosenv.2007.09.013, 2008.
Maher, B. A., Ahmed, I. A. M., Karloukovski, V., MacLaren, D. A., Foulds, P. G., Allsop, D., Mann, D. M. A., Torres-Jardón, R., and Calderón-Garcidueñas, L.: Magnetite pollution nanoparticles in the human brain, P. Natl. Acad. Sci. USA, 113, 10797–10801, https://doi.org/10.1073/pnas.1605941113, 2016.
Mahler, R.: LnProc, 3rd edn., Intern. Congr. Biochem., p. 252, Brussels, 1955.
Mahy, N., Bendahan, G., Boatell, M. Ll., Bjelke, B., Tinner, B., Olson, L., and Fuxe, K.: Differential brain area vulnerability to long-term subcortical excitotoxic lesions, Neuroscience, 65, 15–25, https://doi.org/10.1016/0306-4522(94)00472-h, 1995.
Mahy, N., Prats, A., Riveros, A., Andrés, N., and Bernal, F.: Basal ganglia calcification induced by excitotoxicity: an experimental model characterised by electron microscopy and X-ray microanalysis, Acta Neuropathol., 98, 217–225, https://doi.org/10.1007/s004010051072, 1999.
Maity, R., Venkateshwarlu, M., Mondal, S., Kapawar, M. R., Gain, D., Chatterjee, S., and Paul, P.: Mineral magnetic and geochemical characterization of the dust and soils around Mejia Thermal Power Plant, West Bengal: Implications to source apportionment, Proceedings of the Indian Academy of Sciences, Earth and Planetary Sciences/Journal of Earth System Science, 131, 138, https://doi.org/10.1007/s12040-022-01882-5, 2022.
Mann, S.: Structure, morphology, and crystal growth of bacterial magnetite, in: Magnetite Biomineralization and Magnetoreception in Organisms: A New Biomagnetism, edited by: Kirschvink, J. L., Jones, D. S., and MacFadden, B. J., New York, Plenum, New, 311–332, 1985.
Mann, S., Frankel, R. B., and Blakemore, R. P.: Structure, morphology and crystal growth of bacterial magnetite, Nature, 310, 405–407, https://doi.org/10.1038/310405a0, 1984a.
Mann, S., Moench, T. T., and Williams, R. J. P.: A high-resolution electron microscopic investigation of bacterial magnetite: Implications for crystal growth, Proc. R. Soc. Lond. B., 221, 385–393, 1984b.
Mann, S., Sparks, N. H. C., Walker, M. M., and Kirschvink, J. L.: Ultrastructure, Morphology and Organization of Biogenic Magnetite from Sockeye Salmon, Oncorhynchus Nerka: Implications for Magnetoreception, J. Exp. Biol., 140, 35–49, https://doi.org/10.1242/jeb.140.1.35, 1988.
Marques, M. R. C., Loebenberg, R., and Almukainzi, M.: Simulated Biological Fluids with Possible Application in Dissolution Testing, Dissolution Technologies, 18, 15–28, https://doi.org/10.14227/dt180311p15, 2011.
Martyr, A., Gamble, L. D., Hunt, A., Quinn, C., Morris, R. G., Henderson, C., Allan, L., Opdebeeck, C., Charlwood, C., Jones, R. W., Pentecost, C., Kopelman, M. D., Thom, J. M., Matthews, F. E., and Clare, L.: Differences in trajectories of quality of life according to type of dementia: 6-year longitudinal findings from the IDEAL programme, BMC Medicine, 22, 265, https://doi.org/10.1186/s12916-024-03492-y, 2024.
Massover, W. H.: Ultrastructure of ferritin and apoferritin: A review, Micron, 24, 389–437, https://doi.org/10.1016/0968-4328(93)90005-l, 1993.
May, J., Hickey, M., Triantis, I., Palazidou, E., and Kyriacou, P. A.: Spectrophotometric analysis of lithium carbonate used for bipolar disorder, Biomed. Opt. Express, 6, 1067, https://doi.org/10.1364/boe.6.001067, 2015.
Medviediev, O., Gorobets, O. Y., Gorobets, S. V., and Yadrykhins'ky, V. S.: The prediction of biogenic magnetic nanoparticles biomineralization in human tissues and organs, J. Phys. Conf. Ser., 903, 012002, https://doi.org/10.1088/1742-6596/903/1/012002, 2017.
MESH NCBI – Blood-Air Barrier – MESH: https://www.ncbi.nlm.nih.gov/mesh?Db=mesh&Cmd=DetailsSearch&Term=%22Blood-Air+Barrier%22%5BMeSH+Terms%5D#:~:text=The barrier between capillary blood,MEMBRANE and EPITHELIAL CELL cytoplasm, last access: 16 October 2024.
Michel, F. M., Ehm, L., Antao, S. M., Lee, P. L., Chupas, P. J., Liu, G., Strongin, D. R., Schoonen, M. a. A., Phillips, B. L., and Parise, J. B.: The structure of ferrihydrite, a nanocrystalline material, Science, 316, 1726–1729, https://doi.org/10.1126/science.1142525, 2007.
Mink, J. W.: The basal ganglia: focused selection and inhibition of competing motor programs, Prog. Neurobiol., 50, 381–425, https://doi.org/10.1016/s0301-0082(96)00042-1, 1996.
Möller, W., Häussinger, K., Winkler-Heil, R., Stahlhofen, W., Meyer, T., Hofmann, W., and Heyder, J.: Mucociliary and long-term particle clearance in the airways of healthy nonsmoker subjects, J. Appl. Physiol., 97, 2200–2206, https://doi.org/10.1152/japplphysiol.00970.2003, 2004.
Monfrini, E., Arienti, F., Rinchetti, P., Lotti, F., and Riboldi, G. M.: Brain calcifications: genetic, molecular, and clinical aspects, Int. J. Molec. Sci., 24, 8995, https://doi.org/10.3390/ijms24108995, 2023.
Morabet, R. E.: Effects of outdoor air pollution on human health, in: Elsevier eBooks, https://doi.org/10.1016/b978-0-12-409548-9.11012-7, 2018.
Mouritsen, H.: Long-distance navigation and magnetoreception in migratory animals, Nature, 558, 50–59, https://doi.org/10.1038/s41586-018-0176-1, 2018.
Murat, D., Quinlan, A., Vali, H., and Komeili, A.: Comprehensive genetic dissection of the magnetosome gene island reveals the step-wise assembly of a prokaryotic organelle, P. Natl. Acad. Sci. USA, 107, 5593–5598, https://doi.org/10.1073/pnas.0914439107, 2010.
Navarro-Ciurana, D., Corbella, M., and Meroño, D.: Effects of road dust particle size on mineralogy, chemical bulk content, pollution and health risk analyses, Int. J. Environ. Res. Publ. He., 20, 6655, https://doi.org/10.3390/ijerph20176655, 2023.
Netto, I. and Phutane, V. H.: Reversible lithium neurotoxicity, Primary Care Companion to CNS Disorders/the Primary Care Companion for CNS Disorders, https://doi.org/10.4088/pcc.11r01197, 2012.
Nunomura, A., Perry, G., Aliev, G., Hirai, K., Takeda, A., Balraj, E. K., Jones, P. K., Ghanbari, H., Wataya, T., Shimohama, S., Chiba, S., Atwood, C. S., Petersen, R. B., and Smith, M. A.: Oxidative damage is the earliest event in Alzheimer disease, Journal of Neuropathology and Experimental Neurology, 60, 759–767, https://doi.org/10.1093/jnen/60.8.759, 2001.
Nyirő-Kósa, I., Ahmad, F., Hoffer, A., and Pósfai, M.: Nanoscale physical and chemical properties of individual airborne magnetic particles from vehicle emissions, Atmos. Environ. X, 15, 100181, https://doi.org/10.1016/j.aeaoa.2022.100181, 2022.
Oberdörster, G.: Lung dosimetry: pulmonary clearance of inhaled particles, Aerosol Sci. Technol., 18, 279–289, https://doi.org/10.1080/02786829308959605, 1993.
Oberdörster, G.: Pulmonary effects of inhaled ultrafine particles, Int. Arch. Occ. Env. Hea., 74, 1–8, https://doi.org/10.1007/s004200000185, 2000.
Oberdörster, G. and Kuhlbusch, T. A. J.: In vivo effects: Methodologies and biokinetics of inhaled nanomaterials, NanoImpact, 10, 38–60, https://doi.org/10.1016/j.impact.2017.10.007, 2018.
Ophus, C.: Four-Dimensional scanning Transmission Electron Microscopy (4D-STEM): from scanning nanodiffraction to ptychography and beyond, Microscopy and Microanalysis, 25, 563–582, https://doi.org/10.1017/s1431927619000497, 2019.
O'Shea, M. J., Krekeler, M. P. S., Vann, D. R., and Gieré, R.: Investigation of Pb-contaminated soil and road dust in a polluted area of Philadelphia, Environ. Monitor. A., 193, 440, https://doi.org/10.1007/s10661-021-09213-9, 2021a.
O'Shea, M. J., Toupal, J., Caballero-Gómez, H., McKeon, T. P., Howarth, M. V., Pepino, R., and Gieré, R.: Lead pollution, demographics, and environmental health risks: the case of Philadelphia, USA, Int. J. Environ. Res. Publ. He., 18, 9055, https://doi.org/10.3390/ijerph18179055, 2021b.
O'Shea, M. J., Vigliaturo, R., Choi, J. K., McKeon, T. P., Krekeler, M. P. S., and Gieré, R.: Alteration of yellow traffic paint in simulated environmental and biological fluids, Sci. Total Environ., 750, 141202, https://doi.org/10.1016/j.scitotenv.2020.141202, 2021c.
Ott, M., Stegmayr, B., Renberg, E. S., and Werneke, U.: Lithium intoxication: Incidence, clinical course and renal function – a population-based retrospective cohort study, J. Psychopharmacology, 30, 1008–1019, https://doi.org/10.1177/0269881116652577, 2016.
Outten, F. W. and Theil, E. C.: Iron-Based redox switches in biology, Antioxidants & Redox Signaling, 11, 1029–1046, https://doi.org/10.1089/ars.2008.2296, 2009.
Pankhurst, Q., Hautot, D., Khan, N., and Dobson, J.: Increased levels of magnetic iron compounds in Alzheimer's disease, Journal of Alzheimer's Disease, 13, 49–52, https://doi.org/10.3233/jad-2008-13105, 2008.
Parasrampuria, S., Bijelic, E., Bott, D. M., Driessen, J., Lipp, M. J., and Ling, S. M.: Disaggregating the dementia monolith: An analysis of variation in Medicare costs and use by dementia subtype, Alzheimer S & Dementia, 19, 3295–3305, https://doi.org/10.1002/alz.12953, 2023.
Pecharroman, C., Gonzalez-Carreno, T., and Iglesias, Juan, E.: The infrared dielectric properties of maghemite, γ-Fe2O3, from reflectance measurement on pressed powders, Phys. Chem. Miner., 22, 21–29, https://doi.org/10.1007/bf00202677, 1995.
Piperno, D. R.: Phytoliths. A Comprehensive Guide for Archaeologists and Paleoecologists. ix + 238 pp., AltaMira Press, Rowman & Littlefield, Lanham, New York, Toronto, Oxford, ISBN 0 7591 0385 2, 2006.
Perl, D. P. and Moalem, S.: Aluminum and Alzheimer's disease, a personal perspective after 25 years, Journal of Alzheimer's Disease, 9, 291–300, https://doi.org/10.3233/jad-2006-9s332, 2006.
Plascencia-Villa, G., Ponce, A., Collingwood, J. F., Arellano-Jiménez, M. J., Zhu, X., Rogers, J. T., Betancourt, I., José-Yacamán, M., and Perry, G.: High-resolution analytical imaging and electron holography of magnetite particles in amyloid cores of Alzheimer's disease, Sci. Rep., 6, 24873, https://doi.org/10.1038/srep24873, 2016.
Pósfai, M. and Dunin-Borkowski, R. E.: Magnetic nanocrystals in organisms, Elements, 5, 235–240, https://doi.org/10.2113/gselements.5.4.235, 2009.
Power, A. L., Tennant, R. K., Stewart, A. G., Gosden, C., Worsley, A. T., Jones, R., and Love, J.: The evolution of atmospheric particulate matter in an urban landscape since the Industrial Revolution, Sci. Rep., 13, 8964, https://doi.org/10.1038/s41598-023-35679-3, 2023.
Querol, X., Alastuey, A., Viana, M. M., Rodriguez, S., Artiñano, B., Salvador, P., Santos, S. G. D., Patier, R. F., Ruiz, C. R., De La Rosa, J., De La Campa, A. S., Menendez, M., and Gil, J. I.: Speciation and origin of PM10 and PM2.5 in Spain, J. Aerosol Sci., 35, 1151–1172, https://doi.org/10.1016/j.jaerosci.2004.04.002, 2004.
Quintana, C. and Gutiérrez, L.: Could a dysfunction of ferritin be a determinant factor in the aetiology of some neurodegenerative diseases?, Biochim. Biophys. Ac. G, 1800, 770–782, https://doi.org/10.1016/j.bbagen.2010.04.012, 2010.
Quintana, C., Cowley, J. M., and Marhic, C.: Electron nanodiffraction and high-resolution electron microscopy studies of the structure and composition of physiological and pathological ferritin, J. Struct. Biol., 147, 166–178, https://doi.org/10.1016/j.jsb.2004.03.001, 2004.
Redgrave, P., Prescott, T. J., and Gurney, K.: The basal ganglia: a vertebrate solution to the selection problem?, Neuroscience, 89, 1009–1023, https://doi.org/10.1016/s0306-4522(98)00319-4, 1999.
Reeder, R. J., Schoonen, M. A. A., and Lanzirotti, A.: Metal speciation and its role in bioaccessibility and bioavailability, Rev. Mineral. Geochem., 64, 59–113, https://doi.org/10.2138/rmg.2006.64.3, 2006.
Reusche, E., Pilz, P., Oberascher, G., Lindner, B., Egensperger, R., Gloeckner, K., Trinka, E., and Iglseder, B.: Subacute fatal aluminum encephalopathy after reconstructive otoneurosurgery: A case report, Human Pathology, 32, 1136–1140, https://doi.org/10.1053/hupa.2001.28251, 2001.
Reuzel, P. G. J., Bruijntjes, J. P., Feron, V. J., and Woutersen, R. A.: Subchronic inhalation toxicity of amorphous silicas and quartz dust in rats, Food Chem. Toxicol., 29, 341–354, https://doi.org/10.1016/0278-6915(91)90205-l, 1991.
Roider, G. and Drasch, G.: Concentration of aluminum in human tissues – investigations on an occupationally non-exposed population in Southern Bavaria (Germany), Trace elements and electrolytes, 16, 77-86 0946-210, 1999.
Robertson, D. J., Taylor, K. G., and Hoon, S. R.: Geochemical and mineral magnetic characterisation of urban sediment particulates, Manchester, UK, Appl. Geochem., 18, 269–282, https://doi.org/10.1016/s0883-2927(02)00125-7, 2003.
Ru, Q., Li, Y., Chen, L., Wu, Y., Min, J., and Wang, F.: Iron homeostasis and ferroptosis in human diseases: mechanisms and therapeutic prospects, Signal Transduction and Targeted Therapy, 9, 271, https://doi.org/10.1038/s41392-024-01969-z, 2024.
Salim, S. Y., Kaplan, G. G., and Madsen, K. L.: Air pollution effects on the gut microbiota, Gut Microbes, 5, 215–219, https://doi.org/10.4161/gmic.27251, 2013.
Sanders, T., Liu, Y., Buchner, V., and Tchounwou, P. B.: Neurotoxic effects and Biomarkers of lead exposure: a review, Rev. Environ. Health, 24, 15–46, https://doi.org/10.1515/reveh.2009.24.1.15, 2009.
Sanderson, P., Delgado-Saborit, J. M., and Harrison, R. M.: A review of chemical and physical characterisation of atmospheric metallic nanoparticles, Atmos. Environ., 94, 353–365, https://doi.org/10.1016/j.atmosenv.2014.05.023, 2014.
Schaefer, M. L., Böttger, B., Silver, W. L., and Finger, T. E.: Trigeminal collaterals in the nasal epithelium and olfactory bulb: A potential route for direct modulation of olfactory information by trigeminal stimuli, J. Comp. Neurol., 444, 221–226, https://doi.org/10.1002/cne.10143, 2002.
Schafer, F. Q., Qian, S. Y., and Buettner, G. R.: Iron and free radical oxidations in cell membranes, Cell. Mol. Biol., 46, 657–662, 2000.
Scheiber, I. F., Mercer, J. F. B., and Dringen, R.: Metabolism and functions of copper in brain, Prog. Neurobiol., 116, 33–57, https://doi.org/10.1016/j.pneurobio.2014.01.002, 2014.
Schoonen, M. A. A., Cohn, C. A., Roemer, E., Laffers, R., Simon, S. R., and O'Riordan, T.: Mineral-Induced formation of reactive oxygen species, Rev. Miner. Geochem., 64, 179–221, https://doi.org/10.2138/rmg.2006.64.7, 2006.
Schultheiss-Grassi, P.: TEM investigations of biogenic magnetite extracted from the human hippocampus, Biochim. Biophys. Ac. G, 1426, 212–216, https://doi.org/10.1016/s0304-4165(98)00160-3, 1999.
Semmler-Behnke, M., Takenaka, S., Fertsch, S., Wenk, A., Seitz, J., Mayer, P., Oberdörster, G., and Kreyling, W. G.: Efficient Elimination of Inhaled Nanoparticles from the Alveolar Region: Evidence for Interstitial Uptake and Subsequent Reentrainment onto Airways Epithelium, Environ. Health Persp., 115, 728–733, https://doi.org/10.1289/ehp.9685, 2007.
Shapiro, M.: Plasticity, hippocampal place cells, and cognitive maps, Archives of Neurology, 58, 874, https://doi.org/10.1001/archneur.58.6.874, 2001.
Shirabe, T., Irie, K., and Uchida, M.: Autopsy case of aluminum encephalopathy, Neuropathology, 22, 206–210, https://doi.org/10.1046/j.1440-1789.2002.00432.x, 2002.
Shokrollahi, H.: A review of the magnetic properties, synthesis methods and applications of maghemite, J. Magn. Magn. Mater., 426, 74–81, https://doi.org/10.1016/j.jmmm.2016.11.033, 2017.
Shubin, N., Tabin, C., and Carroll, S.: Deep homology and the origins of evolutionary novelty, Nature, 457, 818–823, https://doi.org/10.1038/nature07891, 2009.
Simonyan, K.: Recent advances in understanding the role of the basal ganglia, F1000Research, 8, 122, https://doi.org/10.12688/f1000research.16524.1, 2019.
Smith, M. A., Harris, P. L. R., Sayre, L. M., and Perry, G.: Iron accumulation in Alzheimer disease is a source of redox-generated free radicals, P. Natl. Acad. Sci. USA, 94, 9866–9868, https://doi.org/10.1073/pnas.94.18.9866, 1997.
Spatz, H.: Über den eisennachweis im gehirn, besonders in zentren des extrapyramidal-motorischen systems. I. Teil, Zeitschrift Für Die Gesamte Neurologie Und Psychiatrie, 77, 261–390, https://doi.org/10.1007/bf02865844, 1922.
Staicu, L., Van Hullebusch, E., and Ackerson, C.: Editorial: Microbial Biominerals: Toward New Functions and Resource Recovery, Front. Microbiol., 12, https://doi.org/10.3389/fmicb.2021.796374, 2021, 2021.
Stefaniak, A. B., Day, G. A., Hoover, M. D., Breysse, P. N., and Scripsick, R. C.: Differences in dissolution behavior in a phagolysosomal simulant fluid for single-constituent and multi-constituent materials associated with beryllium sensitization and chronic beryllium disease, Toxicology in Vitro, 20, 82–95, https://doi.org/10.1016/j.tiv.2005.06.031, 2006.
Steinmetz, J. D., Seeher, K. M., Schiess, N., et al.: Global, regional, and national burden of disorders affecting the nervous system, 1990–2021: a systematic analysis for the Global Burden of Disease Study 2021, Lancet Neurology, 23, 344–381, https://doi.org/10.1016/s1474-4422(24)00038-3, 2024.
Stephens, W. E.: Whewellite and its key role in living systems, Geology Today, 28, 180–185, https://doi.org/10.1111/j.1365-2451.2012.00849.x, 2012.
Stern, A. J., Perl, D. P., Munoz-Garcia, D., Good, P. F., Abraham, C., and Selkoe, D. J.: Investigation of silicon and aluminum content in isolated plaque cores by laser microprobe mass analysis (LAMMA), J. Neuropathol. Exp. Neurol., 45, 361, https://doi.org/10.1097/00005072-198605000-00140, 1986.
Strick, P. L., Dum, R. P., and Fiez, J. A.: Cerebellum and nonmotor function, Annu. Rev. Neurosci., 32, 413–434, https://doi.org/10.1146/annurev.neuro.31.060407.125606, 2009.
Su, R., Jin, X., Li, H., Huang, L., and Li, Z.: The mechanisms of PM2.5 and its main components penetrate into HUVEC cells and effects on cell organelles, Chemosphere, 241, 125127, https://doi.org/10.1016/j.chemosphere.2019.125127, 2020.
Sutherland, R. A.: Lead in grain size fractions of road-deposited sediment, Environ. Pollut., 121, 229–237, https://doi.org/10.1016/s0269-7491(02)00219-1, 2003.
Swift, D. L., Montassier, N., Hopke, P. K., Karpen-Hayes, K., Cheng, Y.-S., Su, N. Y. F., Yeh, N. H. C., and Strong, J. C.: Inspiratory deposition of ultrafine particles in human nasal replicate cast, J. Aerosol Sci., 23, 65–72, https://doi.org/10.1016/0021-8502(92)90318-p, 1992.
Takenaka, S., Karg, E., Roth, C., Schulz, H., Ziesenis, A., Heinzmann, U., Schramel, P., and Heyder, J.: Pulmonary and systemic distribution of inhaled ultrafine silver particles in rats., Environ. Health Persp., 109, 547–551, https://doi.org/10.1289/ehp.01109s4547, 2001.
Tay, A. and Di Carlo, D.: Magnetic Nanoparticle-Based mechanical stimulation for restoration of Mechano-Sensitive ion channel equilibrium in neural networks, Nano Lett., 17, 886–892, https://doi.org/10.1021/acs.nanolett.6b04200, 2017.
Tchounwou, P. B., Yedjou, C. G., Patlolla, A. K., and Sutton, D. J.: Heavy metal toxicity and the environment, in: EXS, 133–164, https://doi.org/10.1007/978-3-7643-8340-4_6, 2012.
Telling, N. D., Everett, J., Collingwood, J. F., Dobson, J., Van Der Laan, G., Gallagher, J. J., Wang, J., and Hitchcock, A. P.: Iron Biochemistry is Correlated with Amyloid Plaque Morphology in an Established Mouse Model of Alzheimer's Disease, Cell Chem. Biol., 24, 1205–1215.e3, https://doi.org/10.1016/j.chembiol.2017.07.014, 2017.
Tingey, A. H.: The iron, copper and manganese content of the human brain, J. Ment. Sci., 83, 452–460, https://doi.org/10.1192/bjp.83.345.452, 1937.
Tingey, A. H.: The Iron Content of the Human Brain. – II, J. Ment. Sci., 84, 980–984, https://doi.org/10.1192/bjp.84.353.980, 1938.
Tisato, F., Marzano, C., Porchia, M., Pellei, M., and Santini, C.: Copper in diseases and treatments, and copper-based anticancer strategies, Med. Res. Rev., 30, 708–749, https://doi.org/10.1002/med.20174, 2009.
Towe, K. M. and Bradley, W. F.: Mineralogical constitution of colloidal “hydrous ferric oxides”, J. Colloid Interf. Sci., 24, 384–392, https://doi.org/10.1016/0021-9797(67)90266-4, 1967.
Treffry, A., Harrison, P. M., Cleton, M. I., De Bruijn, W. C., and Mann, S.: A note on the composition and properties of ferritin iron cores, Journal of Inorganic Biochemistry, 31, 1–6, https://doi.org/10.1016/0162-0134(87)85001-8, 1987.
Treiber, C. D., Salzer, M. C., Riegler, J., Edelman, N., Sugar, C., Breuss, M., Pichler, P., Cadiou, H., Saunders, M., Lythgoe, M., Shaw, J., and Keays, D. A.: Clusters of iron-rich cells in the upper beak of pigeons are macrophages not magnetosensitive neurons, Nature, 484, 367–370, https://doi.org/10.1038/nature11046, 2012.
Trouvé, G., Michelin, L., Kehrli, D., Josien, L., Rigolet, S., Lebeau, B., and Gieré, R.: The Multi-Analytical Characterization of Calcium Oxalate Phytolith Crystals from Grapevine after Treatment with Calcination, Crystals, 13, 967, https://doi.org/10.3390/cryst13060967, 2023.
Tsolaki, E.: Pathological mineralization, Thesis PhD, Department of Medical Physics and Biomedical Engineering University College London, 2020.
Tsolaki, E. and Bertazzo, S.: Pathological mineralization: The potential of mineralomics, Materials, 12, 3126, https://doi.org/10.3390/ma12193126, 2019.
United Nations, Department of Economic and Social Affairs, Population Division: World Urbanization Prospects: The 2018 Revision (ST/ESA/SER.A/420), United Nations, New York, 2019.
United Nations Department of Economic and Social Affairs, Population Division: World Population Prospects 2022: Summary of Results, UN DESA/POP/2022/TR/NO, 3, 2022.
Utembe, W., Potgieter, K., Stefaniak, A. B., and Gulumian, M.: Dissolution and biodurability: Important parameters needed for risk assessment of nanomaterials, Part. Fibre Toxicol., 12, 11, https://doi.org/10.1186/s12989-015-0088-2, 2015.
Vali, H., Kirschvink, J. L., Frankel, R. P., and Blackemore, R. P. (Eds.): Iron biomineralization, Pleum Press, New York, 97, 1991.
Verdoux, H. and Bourgeois, M.: Séquelles neurologiques irréversibles induites par le lithium [Irreversible neurologic sequelae caused by lithium], Encephale, 17, 221–224, PMID: 1864256, 1991 (in French).
Vigliaturo, R., Della Ventura, G., Choi, J., Marengo, A., Lucci, F., O'Shea, M., Pérez-Rodríguez, I., and Gieré, R.: Mineralogical Characterization and Dissolution Experiments in Gamble's Solution of Tremolitic Amphibole from Passo di Caldenno (Sondrio, Italy), Minerals, 8, 557, https://doi.org/10.3390/min8120557, 2018.
Vigliaturo, R., Kehrli, D., Garra, P., Dieterlen, A., Trouve, G., Dietze, V., Wilson, J. P., and Gieré, R.: Opaline phytoliths in Miscanthus sinensis and its cyclone ash from a biomass-combustion facility, Industrial Crops and Products, 139, 111539, https://doi.org/10.1016/j.indcrop.2019.111539, 2019.
Vigliaturo, R., Jamnik, M., Dražić, G., Podobnik, M., Žnidarič, M. T., Della Ventura, G., Redhammer, G. J., Žnidaršič, N., Caserman, S., and Gieré, R.: Nanoscale transformations of amphiboles within human alveolar epithelial cells, Sci. Rep., 12, 1782, https://doi.org/10.1038/s41598-022-05802-x, 2022.
Vigliaturo, R., Jamnik, M., Dražić, G., Podobnik, M., Žnidarič, M. T., Della Ventura, G., Redhammer, G. J., Žnidaršič, N., Caserman, S., and Gieré, R.: Localization and Dimensional Range of Amphibole Particles Retrieved from Human Alveolar Epithelial Cells, Minerals, 14, 101, https://doi.org/10.3390/min14010101, 2024.
Walcott, C., Gould, J. L., and Kirschvink, J. L.: Pigeons have magnets, Science, 205, 1027–1029, https://doi.org/10.1126/science.472725, 1979.
Wang, J., Li, S., Li, H., Qian, X., Li, X., Liu, X., Lu, H., Wang, C., and Sun, Y.: Trace metals and magnetic particles in PM2.5: Magnetic identification and its implications, Sci. Rep., 7, 9865, https://doi.org/10.1038/s41598-017-08628-0, 2017.
Ward, R. J., Zucca, F. A., Duyn, J. H., Crichton, R. R., and Zecca, L.: The role of iron in brain ageing and neurodegenerative disorders, Lancet Neurology, 13, 1045–1060, https://doi.org/10.1016/s1474-4422(14)70117-6, 2014.
Wechsler, B. A., Lindsley, D. H., and Prewitt, C. T.: Crystal structure and cation distribution in titanomagnetites (Fe (sub 3-x) Ti x O 4 ), Am. Mineral., 69, 754–770, 1984.
WHO: Library Cataloguing-in-Publication Data: Evaluation of certain food additives and contaminants: seventy-fourth report of the Joint FAO/WHO Expert Committee on Food Additives, WHO technical report series, no. 966, 2011.
WHO: Global air quality guidelines: Particulate matter (PM2.5 and PM10), ozone, nitrogen dioxide, sulfur dioxide and carbon monoxide, World Health Organization, Geneva, 2021.
Wieland, S., Balmes, A., Bender, J., Kitzinger, J., Meyer, F., Ramsperger, A. F., Roeder, F., Tengelmann, C., Wimmer, B. H., Laforsch, C., and Kress, H.: From properties to toxicity: Comparing microplastics to other airborne microparticles, J. Hazard. Mater., 428, 128151, https://doi.org/10.1016/j.jhazmat.2021.128151, 2022.
Wohlleben, W., Ma-Hock, L., Boyko, V., Cox, G., Egenolf, H., Freiberger, H., Hinrichsen, B., Hirth, S., and Landsiedel, R.: Nanospecific Guidance in REACH: A Comparative Physical-Chemical Characterization of 15 Materials with Methodical Correlations, J. Ceram. Sci. Technol., 04, 93–104, https://doi.org/10.4416/JCST2012-00045, 2013.
Yang, T., Liu, Q., Li, H., Zeng, Q., and Chan, L.: Anthropogenic magnetic particles and heavy metals in the road dust: Magnetic identification and its implications, Atmos. Environ., 44, 1175–1185, https://doi.org/10.1016/j.atmosenv.2009.12.028, 2010.
Yang, Y., Vance, M., Tou, F., Tiwari, A., Liu, M., and Hochella, M. F.: Nanoparticles in road dust from impervious urban surfaces: distribution, identification, and environmental implications, Environ. Sci. Nano, 3, 534–544, https://doi.org/10.1039/c6en00056h, 2016.
Yang, Y., Arseni, D., Zhang, W., Huang, M., Lövestam, S., Schweighauser, M., Kotecha, A., Murzin, A. G., Peak-Chew, S. Y., Macdonald, J., Lavenir, I., Garringer, H. J., Gelpi, E., Newell, K. L., Kovacs, G. G., Vidal, R., Ghetti, B., Ryskeldi-Falcon, B., Scheres, S. H. W., and Goedert, M.: Cryo-EM structures of amyloid-β 42 filaments from human brains, Science, 375, 167–172, https://doi.org/10.1126/science.abm7285, 2022.
Zecca, L., Youdim, M. B. H., Riederer, P., Connor, J. R., and Crichton, R. R.: Iron, brain ageing and neurodegenerative disorders, Nat. Rev. Neurosci., 5, 863–873, https://doi.org/10.1038/nrn1537, 2004.
Zhan, C., Xie, M., Lu, H., Liu, B., Wu, Z., Wang, T., Zhuang, B., Li, M., and Li, S.: Impacts of urbanization on air quality and the related health risks in a city with complex terrain, Atmos. Chem. Phys., 23, 771–788, https://doi.org/10.5194/acp-23-771-2023, 2023.
Zhang, C., Qiao, Q., Piper, J. D. A., and Huang, B.: Assessment of heavy metal pollution from a Fe-smelting plant in urban river sediments using environmental magnetic and geochemical methods, Environ. Pollut., 159, 3057–3070, https://doi.org/10.1016/j.envpol.2011.04.006, 2011.
Zhu, Z., Sun, G., Bi, X., Li, Z., and Yu, G.: Identification of trace metal pollution in urban dust from kindergartens using magnetic, geochemical and lead isotopic analyses, Atmos. Environ., 77, 9–15, https://doi.org/10.1016/j.atmosenv.2013.04.053, 2013.
Zuddas, P., Faivre, D., and Duhamel, J. R.: Magnetite minerals in the human brain: What is their role?, in: Springer eBooks, 91–99, https://doi.org/10.1007/978-94-007-4372-4_6, 2013.
- Abstract
- Introduction
- Anatomy of the human brain
- Minerals and mineraloids occurring in the human brain
- Biomineralization in the human brain and similarities to magnetotactic bacteria
- Exogenous minerals and mineraloid exposure pathways
- Brain diseases related to biogenic and exogenous minerals and mineraloids
- The anthropogenic imprint on the brain mineralogy
- Conclusions
- Appendix A
- Data availability
- Author contributions
- Competing interests
- Disclaimer
- Acknowledgements
- Financial support
- Review statement
- References
- Abstract
- Introduction
- Anatomy of the human brain
- Minerals and mineraloids occurring in the human brain
- Biomineralization in the human brain and similarities to magnetotactic bacteria
- Exogenous minerals and mineraloid exposure pathways
- Brain diseases related to biogenic and exogenous minerals and mineraloids
- The anthropogenic imprint on the brain mineralogy
- Conclusions
- Appendix A
- Data availability
- Author contributions
- Competing interests
- Disclaimer
- Acknowledgements
- Financial support
- Review statement
- References