the Creative Commons Attribution 4.0 License.
the Creative Commons Attribution 4.0 License.
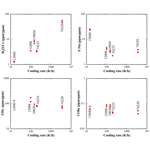
Review of melt inclusions in lunar rocks: constraints on melt and mantle composition and magmatic processes
Youxue Zhang
Mineral-hosted melt inclusions provide a window into magmatic processes and pre-eruptive liquid compositions. Because melt inclusions are small (typically < 100 µm), the study of lunar melt inclusions is enabled by advancements of microbeam instrumental techniques. In the 1970s immediately following the Apollo and Luna missions, major and minor oxide concentrations of lunar melt inclusions were measured using electron microprobes. The data were used to understand magma evolution, and they revealed the immiscibility of two silicate liquids in the late stage of lunar magma evolution. More recently, the development of secondary ion mass spectrometry as well as laser ablation–inductively coupled plasma–mass spectrometry has enabled the measurement of key volatile elements and other trace elements in lunar melt inclusions, down to about the 0.1 ppm level. The applications of these instruments have ushered in a new wave of lunar melt inclusion studies. Recent advances have gone hand in hand with improved understanding of post-entrapment loss of volatiles. These studies have provided deep insights into pre-eruptive volatiles in lunar basalts, the abundance of volatiles in the lunar mantle, the isotopic ratios of some volatile elements, and the partition of trace elements between host olivine and melt inclusions. The recent studies of lunar melt inclusions have played a critical role in establishing a new paradigm of a fairly wet Moon with about 100 ppm H2O in the bulk silicate Moon (rather than a “bone-dry” Moon) and have been instrumental in developing an improved understanding of the origin and evolution of the Moon.
- Article
(2740 KB) - Full-text XML
- BibTeX
- EndNote
A mineral-hosted melt inclusion (MI) is a small pocket (the diameter is typically < 100 µm) of melt entrapped during the growth of the host mineral (Roedder, 1979a). Any mineral can be a host mineral, but some host minerals may protect the melt inclusion against compositional modification better than others, e.g., due to the absence of cleavages and the simplicity of the host composition. A melt inclusion may be glassy, partially glassy, or crystalline depending on the cooling rate of the host mineral (Fig. 1). The importance of MIs is that they can be used to determine the composition of the silicate liquid at the time of host mineral growth, especially the pre-eruptive concentrations of volatiles in the liquid (Johnson et al., 1994). To do so, it is necessary to evaluate various complexities and post-entrapment processes that would modify the composition of an MI compared to the bulk silicate liquid composition from which a mineral grew. The studies of lunar melt inclusions can be divided into two stages. The early stage (1970–1979) immediately followed the Apollo and Luna missions. These investigations focused on the major and minor oxide compositions of the melt inclusions because compositional analyses of MIs require a microbeam technique and at the time the only microbeam technique available was the electron microprobe for major and minor elements. These early studies made an effort to obtain the silicate liquid (melt) composition and evolution, to understand magma evolution, and to document liquid immiscibility in the late stage of lunar magma evolution.
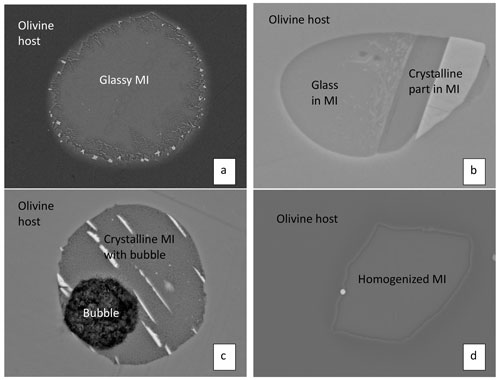
Figure 1Backscattered electron images of some olivine-hosted melt inclusions. (a) A natural glassy MI in olivine in sample 74220 (74220-OL11-MI; Ni et al., 2019). The diameter of the MI is 62 µm. The post-entrapment crystallization (PEC) rim can be seen around the MI as an irregular ∼ 3 µm thick olivine layer with higher Fe concentration (brighter than the surrounding olivine host). There is also PEC of thin dendritic crystals from the MI wall radiating inward. (b) A natural partially glassy MI in olivine in sample 10020 (10020-OL5-NMI; Ni et al., 2019). The glassy part of the MI is labeled and is about 16 µm across. (c) A natural crystalline MI in sample 12008 with a bubble (12008-OL1-NMI; Ni et al., 2019). The MI diameter is 21 µm. (d) A homogenized MI in sample 15016 (15016-OL10-MI; Ni et al., 2019). The size of the MI is 27×42 µm.
In the second stage (2011 and on), melt inclusions are mostly used to determine the concentrations and isotope ratios of volatile and some other trace elements. The new investigations are enabled by advancement in microbeam technology, especially secondary ion mass spectrometry (SIMS) and NanoSIMS, as well as laser ablation–inductively coupled plasma–mass spectrometry (LA-ICP-MS). The Moon is known to be depleted in volatile elements from the first return of Apollo samples (Lunar Sample Preliminary Examination Team, 1969; Gast et al., 1970), but the degree of depletion for each element is of great interest for understanding the origin and evolution of the Moon. For example, the amount of H2O in the bulk silicate Moon (BSM) not only provides constraints on the origin of the Moon (Hartman and Davis, 1975; Cameron and Ward, 1976; Pahlevan et al., 2016; Sharp, 2017; Nakajima and Stevenson, 2018) but also affects the crystallization history of the lunar magma ocean (Elkins-Tanton and Grove, 2011; Lin et al., 2017a, b) and lunar mantle convection (e.g., Goldin, 2014).
This work will review studies on melt inclusions in lunar rocks and what we have learned from such studies.
Most studies of lunar MIs focus on the measurement of MI composition by microbeam methods. Concentrations of major and minor oxides are determined by electron microprobe with a spatial resolution of a couple of micrometers and detection limit approaching 0.01 wt %. Concentrations of non-volatile trace elements are determined by SIMS or LA-ICP-MS with typical spatial resolutions of ≥ 10 µm and detection limits at the parts per million (ppm) to sub-ppm level. Concentrations and isotope ratios of volatile elements in MIs are mostly determined by SIMS and NanoSIMS. The spatial resolution of NanoSIMS can reach the sub-micrometer level, but there is a tradeoff between the beam size and detection limit. A brief description of the analytical methods of electron microprobes, SIMS, and LA-ICP-MS can be found in Cherniak et al. (2010). Most studies of MIs focus on their bulk chemical and isotope composition, which will be reviewed here. However, there are also studies that use concentration gradients in MIs to determine their cooling and evolution history in terrestrial samples (e.g., Newcombe et al., 2014), which have been applied to lunar glass beads (Su et al., 2023) but not to lunar MIs. In addition, there is a significant body of literature on using LA-ICP-MS to measure the bulk composition of unexposed and unhomogenized MIs in terrestrial samples by ablating the whole inclusion and surrounding host and estimating the composition of the MI by deconvolution (e.g., Halter et al., 2002; Pettke et al., 2004; Pettke, 2006; Audetat and Lowenstern, 2014). This method has not been used in the studies of lunar MIs.
Many MIs are analyzed without homogenization experiments, which works well for glassy or partially glassy MIs that have glassy regions for analyses (Fig. 1a and b). The analyzed glass represents the liquid composition of an evolving melt. However, completely glassy or mostly glassy lunar MIs are rare. Many lunar MIs are completely crystalline or have > 50 % crystallinity without large glassy parts for analyses (Fig. 1c). These MIs are heterogeneous, and measurement of these MIs using a microbeam method (rather than the method of ablating the whole MI) may not yield accurate or acceptable bulk composition (Roedder and Weiblen, 1972b). One way to avoid such difficulty is to first pick the host mineral containing MIs (before exposing MIs to the surface) and then heat them to homogenize the MIs inside (Roedder and Weiblen, 1970a, b, 1971; Chen et al., 2015; Ni et al., 2017, 2019). Afterwards, the mineral host is polished to expose the MI for further study. A homogenized MI is shown in Fig. 1d.
There are a number of complexities in using MIs to infer bulk melt compositions. There may be syn-entrapment and post-entrapment modifications. Syn-entrapment modification refers to the possible compositional difference between the melt pocket that is trapped by a growing crystal and the bulk melt composition in the melt reservoir. Post-entrapment modifications include crystallization and bubble growth inside the MI, loss of volatiles due to cryptic decrepitation (MIs with obvious cracks are typically avoided in MI studies), and the exchange of elements with the host and ambient melt. The latter two post-entrapment modifications are not reversible by homogenization experiments. To use melt inclusions to infer original/primitive melt composition, pre-entrapment crystal fractionation and degassing must also be considered. Below, these modifications are explained and discussed in more detail, with a focus on the effect on the concentrations of volatile elements.
- i.
Pre-entrapment degassing. A magma containing high concentrations of volatiles may become oversaturated at shallower depth and experience degassing. For example, a terrestrial basaltic melt containing 2000 ppm CO2 would begin bubble growth and degassing at about 0.4 GPa (Stolper and Holloway, 1988), or more than 10 km depth. All other volatiles would partition into the gas phase depending on its solubility. The gas phase would be completely or partially lost as the magma rises. Hence, pre-entrapment degassing would reduce the concentrations of volatiles and be a problem in the inference of the abundance of volatiles in primitive melts (or in the mantle source). Currently, there is no good way to correct for such effects.
- ii.
Pre-entrapment fractional crystallization. This is almost always present, meaning that MIs rarely represent primitive melt composition. Bulk MIs often represent melt composition along the liquid line of descent during crystallization. The concentrations of volatiles increase during fractional crystallization because volatile elements are often incompatible (meaning the average concentration in the crystalline phases is smaller than that in the melt). Use of analog elemental ratios (see below) would circumvent the problem.
- iii.
Syn-entrapment modification. Various authors have discussed whether MIs represent bulk melt from which the host mineral grew. One concern has been that a melt inclusion is small and is part of the diffusive boundary-layer melt next to a growing crystal. Hence, the boundary-layer melt may have a different composition from the bulk melt from which the host mineral grew. This effect would be greater if the MI were smaller or diffusivity were smaller. Lu et al. (1995) investigated MIs of 50 to 400 µm diameter and concluded that the composition of MIs is indistinguishable from the bulk melt. Audetat and Lowenstern (2014) reviewed investigations after Lu et al. (1995) and concluded that compositions of MIs with diameters > 15 µm represent bulk melt except for extremely slowly diffusing components (e.g., P2O5) and for MIs in sieve-textured crystals.
- iv.
Post-entrapment crystallization (Harris and Anderson, 1983; Kress and Ghiorso, 2004; Newcombe et al., 2014). This is always present even for a completely glassy MI. For a glassy MI, post-entrapment crystallization means the growth of the host mineral inward into the MI. If the host mineral has a simple composition (e.g., olivine), this can be corrected using model calculations by adding the host mineral into the MI to reach equilibrium between the host and the MI (e.g., Harris and Anderson, 1983; Hauri et al., 2011). For crystalline MIs, post-entrapment crystallization includes both host mineral growth into the MI and crystallization in the interior of the MI. If a large enough area is glassy in the MI (Fig. 1b), the glassy part can be analyzed, which represents the composition of the melt along the liquid line of descent. By using analog elemental ratios (see below), the effect of post-entrapment crystallization would be circumvented. Post-entrapment crystallization might also be reversed using homogenization experiments (Roedder et al., 1970a, b, 1971; Chen et al., 2015, 2022), although it is difficult to choose the right temperature, pressure, and duration for the perfect reversal of the post-entrapment crystallization effect (Pettke, 2006). Many crystalline MIs were studied without heating to homogenize them. These MIs contain different mineral (including glass) phases and are hence highly heterogeneous, leading to difficulties in determining the bulk composition of an MI (Roedder and Weiblen, 1972b; Chen et al., 2015).
- v.
Post-entrapment bubble growth (Roedder and Weiblen, 1970a; Wallace et al., 2015). Often a bubble can be found in an MI (e.g., Fig. 1c), which would have changed the concentrations of the volatile elements in the MI. One way to correct for this is to homogenize the MI before analyses, but homogenization may not be perfect (e.g., depending on whether the homogenization temperature, pressure, and duration are correctly chosen; Pettke et al., 2006) and homogenization may cause additional loss of volatiles, especially H2O (Ni et al., 2017). Another way is by model calculations (Wallace et al., 2015).
- vi.
Post-entrapment decrepitation. A melt inclusion may be fractured, leading to leakage of volatiles, often due to pressure decrease outside the MI (Lowenstern, 1995). MIs with obvious cracks are avoided in determining concentrations of volatiles in them. However, cracks may not be obvious (e.g., healed or polished away), but there may still be loss of volatiles due to cryptic decrepitation. Loss of volatiles due to decrepitation is not reversible by homogenization of MIs.
- vii.
Post-entrapment diffusive exchange. This exchange between the melt inclusion and the host crystal as well as the magma around the host mineral may cause loss of volatiles and compositional modification (Qin et al., 1992; Gaetani and Watson, 2000; Chen et al., 2011, 2015; Gaetani et al., 2012; Ni et al., 2017). This process has been theoretically modeled and experimentally measured. The key parameters in this process are the MI radius, the host radius, the position of the MI in the host (an MI is rarely at the center of the host; if an MI were very close to one surface, there would be more diffusive exchange), the diffusivity of the element under consideration, the cooling timescale (or cooling rate), and the partition coefficient of the element between the host mineral and the MI. There has been increased understanding of the timescale for significant post-entrapment diffusive H2O loss from olivine-hosted MIs (OHMIs) with diameters < 40 µm: Hauri (2002), Chen et al. (2011), and Gaetani et al. (2012) concluded that the timescale is very short, about an hour. Ni et al. (2017) concluded that the timescale is even shorter: noticeable loss of H2O can happen in minutes. For other volatiles such as F, S, and Cl, the timescale for their loss is much longer, hours or more (Ni et al., 2017, 2019). For divalent cations, the timescale for noticeable concentration variation due to diffusive exchange with the host and surrounding melt is often years (Gaetani and Watson, 2000). Such diffusive loss or exchange cannot be reversed by homogenization of MIs.
In summary, post-entrapment crystallization and bubble growth can be roughly reversed by homogenization but heating conditions must be chosen well (Ni et al., 2017). Decrepitation and exchange with the host and ambient melt cannot be reversed. Using rapidly quenched and large glassy melt inclusions comprise the best way to prevent post-entrapment loss of H2O. Analog elemental ratios may be used to circumvent the effect of pre-entrapment and post-entrapment crystallization. Cryptic decrepitation would cause loss of volatiles but is expected to be less than prevalent. Hence, it is best to measure several (e.g., ≥ 5) MIs in the same sample and ignore the few data with low concentrations of volatiles. Pre-entrapment degassing is the most difficult to assess and correct. A specific difficulty in studying lunar melt inclusions is the limited number of lunar samples available. When investigating terrestrial problems, one may choose specific samples, such as naturally glassy melt inclusions in submarine basalt, which quenched rapidly in water under “high” pressure (pressure of several hundred bars). Because of the high pressure, such melts may have not suffered from oversaturation of volatiles that would have led to degassing, especially if the melt itself contains low concentrations of volatiles (e.g., due to a high degree of partial melting). Hence, such MIs provide the best samples to infer primitive concentrations of volatiles (Saal et al., 2002). However, there is no ocean on the Moon and there are no rapidly quenched lunar basalts at high pressures. The only known rapidly quenched naturally glassy MIs are found in lunar fire-fountain eruptions on lunar surfaces at near vacuum.
The use of analog elemental ratios, often the ratio of a volatile element to a refractory element, has a long history in geochemical research. For example, Wasserburg et al. (1964) found that the ratio in terrestrial rocks is almost constant, about 10 000. Sun and McDonough (1989) developed the sequence of the degree of elemental incompatibility during mantle partial melting and basalt evolution. Essentially, two elements with a similar degree of incompatibility in magmatic processes are used to form a ratio. Hence, the ratio is roughly constant during mantle partial melting and basalt evolution and can be used to constrain the ratio in the primitive melt and the mantle. The analog element pairs are found empirically (e.g., Wasserburg et al., 1964; Sun and McDonough, 1989; McDonough and Sun, 1995; Salters and Stracke, 2004), and the degree of incompatibility during mantle partial melting can be quantified (Zhang, 2014). The importance of these analog ratios in MI studies is that the effect of pre-entrapment and post-entrapment crystallization can be circumvented by using elemental ratios. For example, Michael (1988, 1995) first found that the ratio is roughly constant in terrestrial MORB (mid-ocean ridge basalts), and Sun and McDonough (1989) showed that F behaves similarly to Nd in MORB. Zhang (2020) showed that and ratios in lunar OHMIs are not affected by up to at least 70 % post-entrapment crystallization, verifying the applicability to lunar magmatic processes. The ratios may be decreased (i.e., the ratios would not be roughly constant) by degassing, bubble growth, diffusive loss, and decrepitation loss of volatiles. In lunar MI inclusion studies, these analog ratios are measured in the glassy part of an MI or in homogenized MI and are examined for whether they are roughly constant. Once the relevant volatile refractory elemental ratio is determined, then the concentration of a volatile element in the bulk silicate Moon (or primitive lunar mantle) can be calculated by multiplying the ratio by the concentration of the denominator refractory element in the bulk silicate Moon. The specific elemental ratios used will be elaborated later.
Even though MIs are our best samples of silicate melt from which the host mineral grew, the interpretation of MIs still requires careful evaluation and necessary correction. For example, as will be clear below, H2O concentrations and ratios in lunar MIs are highly variable and depend on the cooling rate, meaning that MIs in slowly quenched host lost H2O/exchanged H2O with the host and the ambient melt. CO2 (and/or CO) concentration in the lunar mantle is especially difficult to constrain even from MIs due to the low solubility of CO2 (and/or CO) in basalts (e.g., Stolper and Holloway, 1988), due to extensive pre-entrapment and post-entrapment loss through degassing. On the other hand, it appears that pre-eruptive F, S, and Cl concentrations in lunar basalts are well constrained by glassy or homogenized MIs due to their high solubility in melts and small diffusivity in the host mineral (Ni et al., 2017; Zhang, 2020).
Between 1970 and 1979, intensive investigations of lunar samples brought back mostly by the Apollo missions of the USA and some by the Luna missions of the USSR in the space race led to a flurry of publications and discoveries. At the time, the only microbeam analytical technique applied to the studies of small samples of MIs was the electron microprobe for major oxides (≥ 1 wt %, often including SiO2, TiO2, Al2O3, FeO, MgO, and CaO) and minor oxides (0.1 wt % to 1.0 wt %, often including Cr2O3, MnO, Na2O, K2O, and P2O5). The microbeam analyses of trace element and volatile elements became available much later. The investigations focused on the description of the MIs in various host minerals, the analyses of major and minor oxides in the MIs, and interpretation of major oxide evolution trends (liquid line of descent). Silicate liquid immiscibility was discovered in lunar melt inclusions before it was detected in any other natural samples, including terrestrial samples. Heating experiments were used to homogenize the MIs for more accurate analyses of the MI composition (as noted before, post-entrapment exchange and decrepitation volatile loss cannot be reversed by homogenization) and to determine the crystallization sequence.
Roedder and Weiblen (1970a, b) investigated MIs in Apollo 11 samples (high-Ti mare basalts). They observed the presence of two silicate liquids (now glasses) in lunar MIs. That is, they discovered liquid immiscibility in lunar melts. The compositions of the two liquids are roughly potassic granite (also referred to as high-Si liquid) and ferroan pyroxenite (also referred to as the high-Fe liquid). The compositions of two coexisting liquids are somewhat variable, although the variation was deemed to be small by the authors who reported the average compositions. Figure 2 shows the compositional variations in the two liquids measured in these studies and some later studies. Experiments and modeling indicate that the immiscibility occurs late in the magma evolution, at 90 % to 98 % crystallization and temperatures of 1135 to 1075 ∘C. To my knowledge, this was the first time silicate liquid immiscibility was observed in any natural samples, lunar or terrestrial. The authors also experimentally determined the crystallization sequence of lunar sample 10020 (a high-Ti basalt) in the presence of olivine (used as the container): ilmenite at 1210 ∘C, pyroxene at 1140 ∘C, plagioclase at 1105 ∘C, and complete solidification at 1075 ∘C. They reported the major oxide composition of a homogenized MI in 10020 and host olivine measured by electron microprobe. The composition of the homogenized MI in 10020 is similar to the whole-rock composition of 10020.
Roedder and Weiblen (1971) studied MIs in Apollo 11 and Apollo 12 mare basalt samples. They homogenized three OHMIs in 12018. These homogenized MIs are more evolved than the whole-rock composition and are thought to represent melt composition along the liquid line of descent assuming the homogenization condition was chosen well. They also found similar silicate liquid immiscibility in terrestrial basalts such as Hawaii, the Modoc lava field in California, Disko Island in Greenland, and the Precambrian North Shore volcanic group in Minnesota. Silicate liquid immiscibility has also been proposed to explain observed compositions in lunar plutonic rocks formed in lunar crust (Jolliff, 1991; Jolliff et al., 1999).
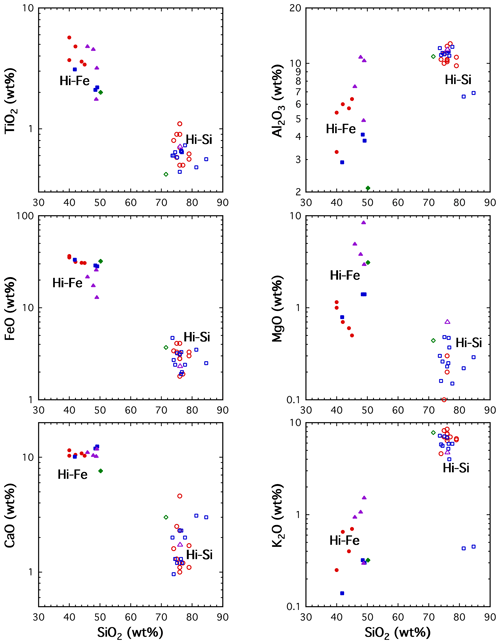
Figure 2Selected compositions of two liquids in silicate liquid immiscibility. Filled symbols are for high-Fe endmembers, and open symbols for high-Si endmembers. Blue squares are for MIs in sample 10050 (Roedder and Weiblen, 1970), green diamonds are for MIs in 10072 (Roedder and Weiblen, 1970), red circles are for MIs in 12057 (Roedder and Weiblen, 1971), and purple triangles are matrix glass in 15343 (Hollister and Crawford, 1977). One-to-one correspondence between the two liquids is not available. Data with concentration < 0.1 wt % are not shown because such electron microprobe data might have large relative errors.
Many later studies (Roedder and Weiblen, 1972a, b, 1973, 1977a, b, 1978; Weiblen and Roedder, 1973, 1976a, b; Weiblen, 1977; Roedder, 1979b) researched MIs in other lunar mare basalt samples, including those from both the Apollo missions and the Luna missions. They provided more detail on these lunar rocks and further established silicate liquid immiscibility (Hollister and Crawford, 1977). Some of these papers made an effort to derive the liquid line of descent for mare basalts (e.g., Weiblen and Roedder, 1976a, b; Roedder and Weiblen, 1977b, c; Weiblen, 1977). However, several complexities compromised this effort; e.g., there are different compositional trends of mare basalts (e.g., high-Ti, low-Ti, and very-low-Ti basalts), most MIs experienced post-entrapment crystallization, and most MIs analyzed by the authors were crystalline without homogenization.
Taylor et al. (1980) discussed the role of silicate liquid immiscibility in the evolution of the lunar magma ocean or in the formation of KREEP. (KREEP rocks are lunar highland basalts with enrichment of K, REE (rare earth elements), and P, thought to represent the last residual melt after crystal fractionation of a lunar magma ocean (LAPST, 1985), or the product of partial melting of lunar highland crust with crystal fractionation (Walker et al., 1972).) They concluded that there was no evidence for extensive silicate liquid immiscibility forming two large-scale physically separated liquids in the evolution of the lunar magma ocean.
These early studies of lunar melt inclusions have contributed to the studies of the Moon and more generally magmatic processes in a number of ways. Silicate liquid immiscibility, first revealed by studies of melt inclusions in lunar rocks, is still a contender for the occurrence of lunar silicic rocks (e.g., Heiken et al., 1991; Siegler et al., 2023). The discovery of silicate liquid immiscibility led to numerous subsequent studies on lunar and terrestrial rocks, including lunar plutonic rocks (Jolliff, 1991; Jolliff et al., 1999), the effect of silicate liquid immiscibility on isotope fractionation (e.g., Kyser et al., 1998), the role of silicate liquid immiscibility in the Daly gap (e.g., Charlier et al., 2011), and the evolution of layered intrusions (e.g., Jakobsen et al., 2005).
The early studies of lunar MIs also explored the experimental homogenization of crystalline melt inclusions as the best ways to investigate the lunar melt composition of major and minor oxides, as well as the crystallization sequence of such melts. In fact, Roedder et al. (1972b) warned that crystalline or partially crystalline MIs without homogenization can make analyses difficult to interpret. Hence, they concluded that homogenization is a good way to obtain more reliable non-volatile element composition of silicate melts.
The advancement of microbeam technology, especially SIMS, ushered in a new stage of studies of lunar samples including lunar melt inclusions, especially the determination of pre-eruptive volatile concentrations of lunar basalts using melt inclusions. These studies led to a paradigm shift from a “bone-dry” Moon to a fairly wet Moon, though not as wet as the Earth. The studies on isotope ratios were often used for comparison with terrestrial isotope ratios to discuss the origin of the Moon, as well as isotope variation during magmatic processes, especially degassing.
4.1 Concentrations of volatiles and concentration ratios of volatile refractory elements
From the Apollo era to about 2007, the lunar interior was thought to be essentially devoid of H2O, sometimes thought to be < 1 ppb (Taylor et al., 2006). Saal et al. (2008) published a paradigm-shifting paper showing that lunar volcanic glass beads contain up to 46 ppm H2O. Because lunar volcanic glass beads must have lost most of their pre-eruptive H2O on the near-vacuum surface of the Moon, there was an intensive search for better lunar samples to obtain the pre-eruptive H2O content of lunar volcanic rocks. Mineral-hosted MIs in lunar basalts are an excellent candidate for such studies because the host mineral provides some protection against the loss of volatile elements.
In addition to reporting the concentrations of volatiles, ratios of , , , , and are often reported to correct for the effect of magma evolution, including pre-entrapment and post-entrapment crystallization. These ratios have been shown empirically to be roughly constant in magmatic processes such as partial melting and fractional crystallization by the study of terrestrial submarine (hence rapidly quenched) samples (Michael, 1988, 1995; Sun and McDonough, 1989; Saal et al., 2002; Workman et al., 2006; Koleszar et al., 2009; Hirschmann, 2016). In other words, the volatile element in the numerator and the refractory element in the denominator for a given ratio have a similar degree of incompatibility during partial melting and crystal fractionation. The denominator element may be referred to as an analog element for the numerator volatile element. Nonetheless, all ratios show some variability. Some ratios are the best found so far but far from perfect. If the two elements were perfect analogs, the ratio in the depleted MORB mantle (DMM) would be identical to that in the primitive Earth mantle or the bulk silicate Earth (BSE). Below I briefly discuss each ratio based on terrestrial MORB and OIB (ocean island basalts) studies and then the applications to lunar samples.
For the ratio, the difference between undegassed submarine and rapidly quenched MORB and OIB is not significant, 100–300 (Michael, 1988, 1995; Dixon and Clague, 2001; Dixon et al., 2002). Chen et al. (2015) and Ni et al. (2019) showed that in lunar sample 10020, the homogenized MI and the glassy parts of partially crystalline MIs (representing ∼ 70 % crystallization) have indistinguishable and ratios (Zhang, 2020), demonstrating that these ratios are not significantly changed by crystal fractionation. Nonetheless, the measured ratio in melt inclusions in lunar basalts varies by 2 orders of magnitude, and the interpretation is that H2O concentration and the ratio can be easily decreased by degassing as well as diffusive exchange with the host and ambient melt (Chen et al., 2011; Ni et al., 2017). Hence, the ratio in melt inclusions with the highest quench rate (lunar sample 74220) is often taken to best represent the ratio in the lunar mantle.
The ratio (Sun and McDonough, 1989) is not much different in MORB (more depleted terrestrial mantle) and OIB (less depleted terrestrial mantle). The ratio is inferred to be 15.4 in DMM (Salters and Stracke, 2004) and 20 in BSE (McDonough and Sun, 1995), with only a small difference. Hence, Nd is a good analog of F except for degassing. An even better analog ratio is (0.270 in DMM and 0.278 in BSE; Salters and Stracke, 2004; McDonough and Sun, 1995), but P has a lower condensation temperature than Nd (Lodders, 2003; Wood et al., 2019).
The ratio (e.g., Saal et al., 2002; Koleszar et al., 2009) varies somewhat between MORB and OIB. The inferred ratio is 224 in DMM and 371 in BSE, indicating that S is somewhat more incompatible than Dy. However, S partition behavior becomes very different when sulfide melt forms, which is fairly common in lunar basalts (Heiken et al., 1991). Hence, it is necessary to use more primitive lunar basalts with higher ratios when estimating the pre-eruptive ratio in lunar basalts.
For Cl, a ratio often used is (Saal et al., 2002; Chen et al., 2015; Shimizu et al., 2016). The ratio increases significantly from MORB (Saal et al., 2002) to OIB (Workman et al., 2006; Koleszar et al., 2009; Sides et al., 2014; see also Chen et al., 2015). The inferred ratio is 0.425 in DMM (Salters and Stracke, 2004) and 2.58 in BSE (McDonough and Sun, 1995), a difference of a factor of 6. Hence, Cl is much more incompatible than Ba during mantle partial melting. There is no perfect analog element for Cl.
For CO2, the ratio is often used (Rosenthal et al., 2015; Hirschmann et al., 2016). The inferred ratio is variable, 42 to 133 in DMM (Salters and Stracke, 2004; Rosenthal et al., 2015) and 67 in BSE (McDonough and Sun, 1995), values which are not too different. A complication is that CO2 has very low solubility in basaltic melts and hence may begin degassing at great depth before MI entrapment. Hence, pre-eruptive CO2 concentrations are difficult to obtain even in terrestrial basalts and much more difficult to obtain in lunar basalts due to limited sampling as well as high vacuum of the lunar surface.
The advantages of using ratios of analog elements rather than concentrations include the following: (1) pre-entrapment and post-entrapment crystallization does not need to be corrected; (2) the ratios obtained for pre-eruptive basalts (that is, pre-degassing basalts) are close to mantle source ratios and may be used to constrain the concentration of the volatile element in the bulk silicate Moon (BSM) if that of the refractory element is estimated independently. Nonetheless, such ratios can still decrease if an MI is near a crack or a surface, if there was degassing or decrepitation, or if there was diffusive loss due to slow post-eruptive cooling. Studies show that whether lunar MIs preserve pre-eruptive elemental ratios depends on the specific volatile component. Based on the small variability in , , and ratios in lunar MIs (Chen et al., 2015; Ni et al., 2019), as well as heating experiments (Ni et al., 2017), the lunar MIs seem to provide rather pristine , , and ratios after removing obvious low ratios. However, the ratio in studied lunar MIs varies by 2 orders of magnitude (Chen et al., 2015; Ni et al., 2019), and heating experiments show that there is significant H2O loss in MIs in just minutes, especially for small MIs (Ni et al., 2017) (Fig. 3). The results are understandable because H2O has high diffusivity in the host (Demouchy and Mackwell, 2006; Ni et al., 2017), and it is easier for smaller MIs to lose volatiles (e.g., Qin et al., 1992; Ni et al., 2017). Hence, lunar MIs do not necessarily provide pristine H2O contents of lunar samples. Instead, the large variation in the ratio was found to depend on the post-eruptive cooling rate, attributed to post-entrapment H2O loss for slowly cooled MIs. That is, the lunar samples documented by Chen et al. (2015), Ni et al. (2019), and Hu et al. (2021) do not directly provide pristine H2O contents of lunar magmas, and much effort is needed to interpret the results. The interpretation is that the most rapidly quenched glassy MIs provide the best pre-eruptive concentrations and ratios. The CO2 concentration in OHMIs in 74220 (not enough data for the ratio) also decreases with decreasing MI diameter (Wetzel et al., 2015; Zhang, 2020). Hence, in the following discussion and plots, often the highest ratios in MIs in a given lunar sample are used, which removes much scatter in the data. For the numerous terrestrial samples shown for comparison, no similar filter is applied and hence sometimes more scatter is present. Below, specific investigations of lunar MIs are discussed.
Hauri et al. (2011, 2015) investigated naturally nearly glassy OHMIs in lunar sample 74220 and discovered H2O concentrations of up to 1410 ppm, F concentrations of up to 78 ppm, Cl concentrations of up to 3.0 ppm, and S concentrations of up to 935 ppm. Hauri et al. (2015) used the data to model the composition of the lunar primitive mantle.
Ni et al. (2017) investigated whether homogenization experiments would cause loss of volatiles from melt inclusions. They applied the homogenization condition of 1330 ∘C, 1 bar, and 2 min to olivine hosts with MIs in 74220. The OHMIs in 74220 are essentially glassy before homogenization and have been well characterized without homogenization (Hauri et al., 2011, 2015; Chen et al., 2015). The purpose of the study was to determine possible loss of volatiles by comparing the concentrations of volatile elements and ratios of volatile refractory elements in homogenized versus natural glassy OHMIs in 74220. Ni et al. (2017) found that for F, Cl, and S, there is no loss due to laboratory homogenization for MI diameters down to ∼ 10 µm. However, after homogenization experiments there is a significant H2O concentration decrease as the diameter of the MI decreases. That is, there is noticeable loss of H2O in small MIs due to homogenization (Fig. 3), even though the heating duration is only about 2 min. The loss of H2O becomes negligible when the diameter of the OHMI is > 60 µm (Fig. 3). In addition, Fig. 3 shows that the ratio in naturally glassy OHMIs in 74220 also decreases with MI diameter, though laboratory-homogenized MIs display additional decrease in the ratio. That is, even for the most rapidly cooled lunar sample, H2O in OHMIs is still partially lost from the smaller MIs. The results show the easiness of H2O loss from OHMIs and the difficulty of constraining the ratio in pre-eruptive lunar basalts and in the lunar mantle. Chen et al. (2015), Ni et al. (2019), and Zhang (2020) concluded that the highest ratio of 50 to 70 found in 74220 best represents the lunar mantle, and the true ratio in the lunar mantle might be even higher.
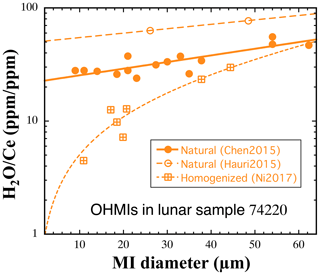
Figure 3The ratio versus diameter of OHMIs in lunar sample 74220. Data are from the following: naturally nearly glassy OHMIs reported by Chen et al. (2015), Hauri et al. (2015), and Ni et al. (2019) and homogenized OHMIs by Ni et al. (2017) at 1330 ∘C and 1 bar for 2 min. Note that the ratios reported by Hauri et al. (2015) (open circles) are systematically higher than those by Chen et al. (2015) (filled circles) and Ni et al. (2019). The systematic difference is attributed mostly to interlaboratory difference in the calibration for H2O. For consistency, data by Chen et al. (2015) and Ni et al. (2017, 2019) from the same laboratory are compared. Adapted from Zhang (2020).
Chen et al. (2015) and Ni et al. (2019) reported volatile and trace element data in OHMIs in sample 74220 and six other lunar samples (10020, 12008, 12040, 15016, 15647, and 74235) and used the volatile refractory elemental ratio approach. Naturally glassy MIs are mostly found in 74220 because this sample had the highest post-eruptive cooling rate compared to other lunar samples that have OHMIs. In the other lunar samples, OHMIs are completely to partially crystalline due to slower post-eruptive cooling. Chen et al. (2015) reported volatile and trace element concentrations in crystalline MIs without homogenization but decided that the data were too scattered to be useful. They then homogenized the crystalline MIs by laboratory heating so that volatile refractory elemental ratios can be determined more reliably (Chen et al., 2015). In addition, glassy parts of OHMIs in 10020 and interstitial glass in 74235 were also analyzed for volatile and trace elements. Chen et al. (2015) and Ni et al. (2017, 2019) found that , , and ratios do not vary much between different lunar samples from glassy to homogenized OHMIs, but the ratio shows a variability of 2 orders of magnitude and is much higher in OHMIs in 74220 than in OHMIs of all other lunar samples (Figs. 4 and 5). The ratio in OHMIs is positively correlated with the post-eruptive quench rate of the volcanic sample, whereas , , and ratios are not (Fig. 5). (The high ratio in one slowly cooled lunar sample (12040) does not yet have a good explanation.) The positive correlation between the ratio and the post-eruptive quench rate implies that slowly cooled samples lost H2O, and the sample with the highest cooling rate (74220) best represents the pre-eruptive H2O concentration and ratio in lunar basalts. Hence, the ratio in lunar basalts is inferred to be about 50 to 70, lower than the ratio in terrestrial MORB and OIB by a factor of 3. Taking Ce concentration in BSM to be 1.675, the same as in the BSE (McDonough and Sun, 1995; Hauri et al., 2015), a ratio of 60±10 implies an H2O concentration of about 100 ppm in the BSM (or primitive lunar mantle) (Chen et al., 2015; Ni et al., 2019; Zhang, 2020).
Hu et al. (2021) studied H2O concentrations and hydrogen isotope ratios in ilmenite-hosted melt inclusions in lunar regolith returned by the Chang'e-5 mission. These melt inclusions are largely crystalline and were not homogenized. They found highly variable H2O concentrations, as expected from crystalline melt inclusions. After PEC correction, they reported H2O concentrations of 6 to 370 ppm in the MIs. These concentrations fall within the range of H2O concentrations in slowly cooled crystalline or partially crystalline OHMIs in Apollo samples. Hu et al. (2021) then made an effort to infer the pre-eruptive H2O concentration using the MI with the highest H2O concentration and lowest ratio and then further tried to infer H2O concentration in the lunar mantle of the Chang'e-5 landing site. As discussed above, the use of slowly cooled MIs in a single sample to estimate pre-eruptive H2O concentration or source mantle composition is not deemed reliable.
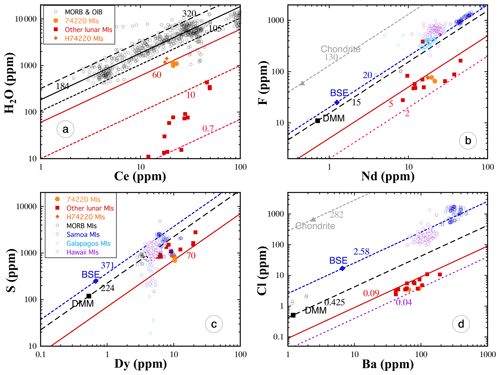
Figure 4Plot of volatile elements versus their refractory analog on a logarithmic scale. Data from lunar MIs are filled orange or red symbols. Terrestrial data (open symbols) are shown for comparison. Lines of constant ratios are shown in each figure. For data from lunar MIs, the highest values from each sample are chosen to avoid the effect of potential loss. For the numerous terrestrial data, no filter is applied. The ratio in lunar MIs varies by 2 orders of magnitude (from about 0.7 to 70). Each of the other ratios in lunar MIs has a limited range. The ratio is mostly between 2 and 5 although one sample (12040) has an outlier ratio of up to 25. The ratio is between 70 and 200. The ratio is between 0.04 and 0.09. Data sources: H74220 data are from Hauri et al. (2015), and other lunar MI data (including 74220 MIs) are from Chen et al. (2015) and Ni et al. (2019). Terrestrial data are from Michael (1988, 1995), Danyushevsky et al. (2000), Dixon and Clague (2001), Dixon et al. (2002), Saal et al. (2002), Workman et al. (2006), and Koleszar et al. (2009). Adapted from Chen et al. (2015) and Ni et al. (2019) with filtering of lunar data to simplify.
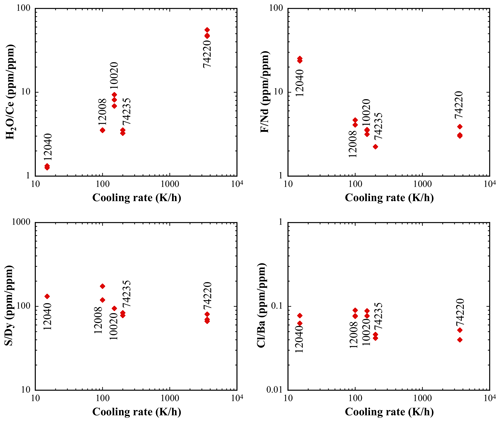
Figure 5The volatile refractory element ratios versus cooling rate for lunar MIs. The highest ratios from each sample are chosen to avoid the effect of the potential loss of volatiles. Note the good correlation between the ratio and cooling rate. The ratio is mostly between 2 and 5 although one sample (12040) is an outlier with a ratio of up to 25. The ratio is between 70 and 200. The ratio is between 0.04 and 0.09. For the ratio, only limited data on one lunar sample (74220) are available, and hence no plot is made. Data sources for ratios: Chen et al. (2015) and Ni et al. (2019). Data source for post-eruptive cooling rates: Donaldson et al. (1975), Usselman et al. (1975), Arndt and Rombach (1976), Ni et al. (2017), and Zhang (2020). The figure is adapted from Zhang (2020).
Wetzel et al. (2015) determined carbon concentrations in OHMIs and glass beads in 74220 using SIMS. This is the only paper reporting carbon concentrations in OHMIs in any lunar samples. They found the ppm level of C in MIs (note that the CO2 concentration is 3.66 times that of C) and a ratio of ∼ 0.07. The concentration of C in lunar basalt is often not calculated as CO2 concentration because there may be significant CO and other carbon species. Wetzel et al. (2015) then corrected for CO2 in bubbles and estimated carbon concentration to be about 10 times higher than in the glassy part of OHMIs, 44–64 ppm, within the range of C concentration in terrestrial MORB (Saal et al., 2002). Nonetheless, due to significantly higher Ba concentration in 74220 glass than in the MORB samples investigated by Saal et al. (2002), even the corrected ratio (∼ 0.7, equivalent to a ratio of 2.6) is still much lower than the inferred ratio of ∼ 133 in the Earth (Rosenthal et al., 2015) by a factor of about 50. Because CO2 degassing starts at relatively high pressure (or depth) and because of the uncertainty in carbon speciation, the ratios measured in OHMIs in lunar volcanic rocks are likely still too low. That is, the ratio in the lunar mantle is likely higher than 2.6.
In summary, except for H2O and C, other volatiles are not significantly lost from MIs during natural cooling or short-duration laboratory heating, and homogenized MIs from slowly cooled samples also provide reliable data for F, S, and Cl. Hence, concentrations of moderately volatile elements such as Zn, Pb, Cs, Rb, Ga, and Cu determined in OHMIs likely represent pre-eruptive concentrations (Ni et al., 2019). Limited whole-rock data on F, S, and Cl measured in the 1970s (Wanke et al., 1975, 1976), though uncertain in terms of their degree of volatile loss, are also in agreement with the new MI data, as shown by Zhang (2020).
The investigation of volatiles in lunar melt inclusions not only expanded the data on lunar volatiles, but also further constrained volatile refractory elemental ratios, especially for and . For the best-constrained volatiles (H2O, F, and S), the new data suggest that these highly volatile elements in the BSM are depleted relative to the BSE by roughly a constant factor of 2 to 5 (Zhang, 2020), independent of the condensation temperature of the volatile elements. One possible explanation for this is that these volatiles were delivered to both the Earth and the Moon by an “early veneer” right after Moon formation, and the Moon received proportionally less of the early veneer planetesimals (Zhang, 2020). The discovery of fairly high concentrations of volatiles, especially H2O, in the Moon also led to the proposals of newer versions of the giant-impact hypothesis for the origin of the Moon (Sharp, 2017; Nakajima and Stevenson, 2017) and the evolution of the Moon (Lin et al., 2017a, b).
4.2 Isotope ratios in lunar MIs
In addition to the abundance of volatile elements in the Moon compared to the Earth, isotope ratios of volatile and moderately volatile elements also provide important constraints on the origin and magmatic evolution of the Moon. Isotopes ratios in MIs are measured in situ by SIMS or NanoSIMS.
Saal et al. (2013) reported H isotope ratios in OHMIs in 74220 as well as lunar volcanic glass beads. There are substantial variations in δD in OHMIs (+187 ‰ to +311 ‰) and many more variations in volcanic glass beads (−733 ‰ to +4045 ‰), largely attributed to degassing. The MIs provide the best pre-eruptive isotope ratio. Saal et al. (2013) concluded that pre-eruptive lunar hydrogen isotopes (or ratios in the lunar mantle) are broadly similar to chondrites and the Earth. Singer et al. (2017) and Stephant et al. (2020) measured H isotope ratios in OHMIs and PHMIs (pyroxene-hosted MIs) in more Apollo mare basalts and reached a similar conclusion.
Stephant et al. (2019) investigated Cl concentration and the isotope ratio in melt inclusions in five mare basalts and compared the results with Cl isotopes in lunar apatite or whole rocks. Cl concentrations in PHMIs are on average 3.6 times those in OHMIs, consistent with magma evolution and later crystallization of pyroxene than olivine. They found OHMIs and pyroxene-hosted MIs have similar ratios, +12.8 ± 2.4 ‰ and +10.1 ± 3.2 ‰, indicating no significant isotope fractionation during magma evolution. These isotope ratios with 37Cl enrichment are roughly similar to those in lunar apatite (Boyce et al., 2015; Barnes et al., 2016). Hence, Stephant et al. (2019) concluded that the enrichment of 37Cl in lunar basalts (+11 ‰) relative to terrestrial basalts (δ37Cl = −1.0 ± 0.9 ‰; Bonifacie et al., 2008) is not due to degassing of anhydrous magma during magma emplacement but is consistent with magma degassing during lunar magma ocean crystallization, leading to a KREEP endmember with high , which then mixed into younger magmas (Boyce et al., 2015).
Saal and Hauri (2021) investigated the S isotope ratio () in OHMIs in 74220, orange glass beads in 74220, and green glass beads in 15426. They found that δ34S values (−0.3 ‰ to +1.6 ‰) in OHMIs in 74220 are similar to previous data in whole-rock samples, but the glass beads, especially the green glass beads in 15426, show lower values with large variability in δ34S down to −14.5 ‰. The variable δ34S is correlated with S concentration and is consistent with degassing of S, leading to increasingly lower δ34S in the remaining melt. It is not resolved whether or not BSM and BSE share a common δ34S.
4.3 Partitioning of elements between olivine and melt
Partition coefficients between minerals and melt have wide applications in understanding and modeling magmatic processes. Melt inclusions have been used to investigate partition coefficients in terrestrial magmas (e.g., Nikogosian and Sobolev, 1997; Thomas et al., 2002). Chen et al. (2022) investigated element partitioning between the olivine host and MIs in lunar basalts. One of the keys in such studies is to ensure that the specific MI is in rough chemical equilibrium with the host mineral. Equilibrium is assessed using the exchange coefficient . This coefficient is about 0.3 (Roeder and Emslie, 1970), but it decreases with increasing TiO2 content in lunar basalt (Xirouchakis et al., 2001). Chen et al. (2022) used the criterion that the measured KD value between the host olivine and the given MI is within 20 % of the experimentally determined TiO2-adjusted KD value.
Naturally glassy MIs in 74220 (Hauri et al., 2011, 2015; Chen et al., 2015; Ni et al., 2019) are not in equilibrium with their host olivine using the KD criterion, presumably due to post-entrapment crystallization on the wall of the host mineral. Chen et al. (2022) evaluated all available melt inclusion and host olivine composition data and selected homogenized MIs that are in rough equilibrium with their host olivine based on TiO2-adjusted KD values. For these MIs, homogenization experiments roughly reversed the post-entrapment crystallization on the host wall and in the interior of the MI. These host–MI pairs are used to determine the partition coefficients of elements between olivine and melt. It was found that for most elements, the partition coefficients between olivine and lunar basaltic melt are similar to those in terrestrial basalts. However, for V and Cr, the partition coefficients in lunar basalts are significantly different from those in terrestrial basalts. The different behavior of these two elements between lunar and terrestrial basalts can be attributed to the lower oxygen fugacity in lunar basalts (about IW − 1; Sato, 1971, 1973; Wadhwa, 2008) by about 4 orders of magnitude compared to terrestrial basalts (about QFM; e.g., O'Neill et al., 2018) and the dependence of the valence of V and Cr on the oxygen fugacity. Hence, Cr is highly compatible during terrestrial basalt evolution but fairly incompatible during lunar basalt evolution. On the other hand, V is more incompatible during terrestrial basalt evolution but less incompatible in lunar basalt evolution. Therefore, these two elements behave very differently in terrestrial magmatic processes but similarly in lunar magmatic processes as a coincidence.
4.4 Other studies of lunar melt inclusion
The work on liquid immiscibility using lunar MIs (e.g., Roedder and Weiblen, 1970a, b, 1971, 1972a, b) has also continued. Zeng et al. (2020) investigated the major and trace element composition of MIs hosted by the oldest zircon (4.382 ± 0.040 Ga) known, in lunar rock NWA10049. They found silica-rich melt in these MIs, which is compositionally similar to the immiscible high-Si melt as documented by Roedder and Weiblen (1970, 1971) but extends to even higher SiO2 (up to 93 wt % SiO2). Zeng et al. (2020) did not report finding the immiscible high-Fe endmember but interpreted the silicic melts to be due to silicate liquid immiscibility. The zircon crystal is 4.38 Ga, older than mare basalts (up to 4.2 Ga; Papike et al., 1998). Zeng et al. (2020) concluded that these comprise the oldest immiscible high-Si melt on the Moon.
Studies of lunar melt inclusions have contributed significantly to understanding of the origin, geochemical evolution, and magmatic processes of the Moon. Progress has often been enabled by advancement in microbeam instrumental methods.
-
The early studies following the Apollo and Luna missions in the 1970s focused on major and minor oxides in lunar melt inclusions. These studies provided constraints on the liquid composition of lunar basalts and especially revealed the occurrence of two-silicate-liquid immiscibility in the late stage of lunar magma evolution. Interestingly, the natural occurrence of silicate liquid immiscibility was first identified in lunar rocks and later identified in terrestrial rocks. Later studies concluded that large-scale silicate liquid immiscibility did not play a major role in lunar magma ocean evolution.
-
More recent research of lunar melt inclusions since 2010 has focused on volatile and trace element concentrations and isotope ratios, fueled by the new discovery that the Moon is not as dry as thought before. These investigations carefully evaluated possible loss of volatiles during cooling of natural MIs as well as during homogenization experiments. They constrained and confirmed the various volatile refractory elemental ratios in the Moon, including (50 to 70), (2 to 5), (70 to 200), and (0.04 to 0.09). The ratio in the Moon is > 0.7. The melt inclusion studies also provided the most reliable pre-eruptive isotope ratios of some volatile elements, including δD, δ37Cl, and δ34S. The new data from MIs in lunar basalts have been instrumental in shifting the paradigm from a bone-dry Moon to a fairly wet Moon. Moreover, they provide important constraints on the composition, origin, and evolution of the Moon.
-
The lunar samples studied so far for abundance of volatiles in MIs are limited: eight in total – 10020, 12008, 12040, 15016, 15647, 74220, 74235, and CE5 (Hauri et al., 2011, 2015; Chen et al., 2015; Wetzel et al., 2015; Ni et al., 2017, 2019; Hu et al., 2021). More samples need to be investigated to further establish how H2O concentration varies in melt inclusions with post-eruptive quench rates (Chen et al., 2015; Ni et al., 2019; Zhang, 2020) and whether and how volatile refractory concentration ratios depend on types of lunar basalts (Ni et al., 2019).
-
It has been shown that except for the most volatile elements (such as H, C, N, and noble gases), MIs (including naturally glassy and homogenized crystalline MIs) preserve pre-eruptive concentrations well. Hence, MIs (glassy or homogenized) are expected to provide reliable concentrations of other volatile elements, such as Hg, Tl, I, In, Br, Cd, Se, Sn, Te, Bi, Ge, B, Sb, Ag, Au, and As, especially if appropriate concentration ratios can be used for the estimation of abundance of volatile elements in BSM. These elements have not been measured in lunar melt inclusions using SIMS or LA-ICP-MS due to their low concentrations, low sensitivity, interference, and/or inadequate glass standards (e.g., Jenner and Arevalo, 2016). Future improvement in instrumentation and standards will be critical for the analyses of these elements.
-
Because homogenization of melt inclusions takes effort, may not completely reverse post-entrapment crystallization and growth, and may cause additional loss of volatiles, it will be desirable to apply the method of LA-ICP-MS to ablate the whole melt inclusion and analyze the composition of the whole MI (e.g., Halter et al., 1995; Pettke et al., 2004; Pettke, 2006). In addition to applications to crystalline MIs, this method may also be applied to MIs smaller than 10 µm.
Data in figures are available upon request.
The author has declared that there are no competing interests.
Publisher’s note: Copernicus Publications remains neutral with regard to jurisdictional claims made in the text, published maps, institutional affiliations, or any other geographical representation in this paper. While Copernicus Publications makes every effort to include appropriate place names, the final responsibility lies with the authors.
This article is part of the special issue “Probing the Earth: Melt and solid inclusions as probes to understand nature”. It is not associated with a conference.
This work is supported by NASA grants 80NSSC19K0782 and 80NSSC23K1297. I thank Peng Ni for taking the SEM photos in Fig. 1 and Thomas Pettke and the anonymous reviewer for their constructive and insightful comments.
This research has been supported by the National Aeronautics and Space Administration (grant nos. 80NSSC19K0782 and 80NSSC23K1297).
This paper was edited by Matteo Alvaro and reviewed by Thomas Pettke and one anonymous referee.
Arndt, J. and Rombach, N.: Derivation of the thermal history of tektites and lunar glasses from their thermal expansion characteristics, Proc. Lunar Sci. Conf. 7th, 1123–1141, 1976.
Audetat, A. and Lowenstern, J. B.: Melt inclusions, Treat. Geochem., 13, 143–173, 2014.
Barnes, J. J., Tartese, R., Anand, M., McCubbin, F. M., Neal, C. R., and Franchi, I. A.: Early degassing of lunar urKREEP by crust-breaching impact(s), Earth Planet. Sc. Lett., 447, 84–94, 2016.
Bonifacie, M., Jendrzejerski, N., Agrinier, P., Humler, E., Coleman, M., and Javoy, M.: The chlorine isotope composition of Earth's mantle, Science, 319, 1518–1520, 2008.
Boyce, J. W., Treiman, A. H., Guan, Y., Ma, C., Eiler, J. M., Gross, J., Greenwood, J. P., and Stolper, E. M.: The chlorine isotope fingerprint of the lunar magma ocean, Sci. Adv., 1, e1500380, https://doi.org/10.1126/sciadv.1500380, 2015.
Cameron, A. G. W. and Ward, W. R.: The origin of the moon, Lunar Planet. Sci. Conf. 7th, 120–122, 1976.
Charlier, B., Namur, O., Toplis, M. J., Schiano, P., Cluzel, N., Higgins, M. D., and Auwera, J. V.: Large-scale silicate liquid immiscibility during differentiation of tholeiitic basalt to granite and the origin of the Daly gap, Geology, 39, 907–910, 2011.
Chen, S., Ni, P., Zhang, Y., and Gagnon, J.: Trace element partitioning between olivine and melt in lunar basalts, Am. Mineral., 107, 1519–1531, 2022.
Chen, Y., Provost, A., Schiano, P., and Cluzel, N.: The rate of water loss from olivine-hosted melt inclusions, Contrib. Mineral. Petrol., 162, 625–636, 2011.
Chen, Y., Zhang, Y., Liu, Y., Guan, Y., Eiler, J. M., and Stolper, E. M.: Water, fluorine, and sulfur concentrations in the lunar mantle, Earth Planet. Sc. Lett., 427, 37–46, 2015.
Cherniak, D. J., Hervig, R. L., Koepke, J., Zhang, Y., and Zhao, D.: Analytical methods in diffusion studies, Rev. Mineral. Geochem., 72, 107–170, 2010.
Danyushevsky, L. V., Eggins, S. M., Falloon, T. J., and Christie, D. M.: H2O abundance in depleted to moderately enriched mid-ocean ridge magmas; Part I: incompatible behavior, implications for mantle storage, and origin of regional variations, J. Petrol., 41, 1329–1364, 2000.
Demouchy, S. and Mackwell, S.: Mechanisms of hydrogen incorporation and diffusion in iron-bearing olivine, Phys. Chem. Minerals, 33, 347–355, 2006.
Dixon, J. E. and Clague, D. A.: Volatiles in basaltic glasses from Loihi seamount, Hawaii: evidence for a relatively dry plume component, J. Petrol., 42, 627–654, 2001.
Dixon, J. E., Leist, L., Langmuir, C., and Schilling, J. G.: Recycled dehydrated lithosphere observed in plume-influenced mid-ocean-ridge basalt, Nature, 420, 385–389, 2002.
Donaldson, C. H., Usselman, T. M., Williams, R. J., and Lofgren, G. E.: Experimental modeling of the cooling history of Apollo 12 olivine basalts, Proc. Lunar Sci. Conf. 6th, 843–869, 1975.
Elkins-Tanton, L. T. and Grove, T. L.: Water (hydrogen) in the lunar mantle: results from petrology and magma ocean modeling, Earth Planet. Sc. Lett., 307, 173–179, 2011.
Gaetani, G. A. and Watson, E. B.: Open system behavior of olivine-hosted inclusions, Earth Planet. Sc. Lett., 183, 27–41, 2000.
Gaetani, G. A., O'Leary, J. A., Shimizu, N., Bucholz, C. E., and Newville, M.: Rapid reequilibration of H2O and oxygen fugacity in olivine-hosted melt inclusions, Geology, 40, 915–918, 2012.
Gast, P. W. and Hubbard, N. J.: Abundance of alkali metals, alkaline and rare earths, and srtontium-87/strontium-86 ratios in lunar samples, Science, 167, 485–487, 1970.
Goldin, T.: Water and the lunar dynamo, Nat. Geosci., 7, p. 400, 2014.
Halter, W. E., Pettke, T., and Heinrich, C. A.: Major to trace element analysis of melt inclusions by laser-ablation ICP-MS: methods of quantification, Chem. Geol., 183, 63–86, 2002.
Harris, D. M. and Anderson, A. T.: Concentrations, sources, and losses of H2O, CO2, and S in Kilauean basalt, Geochim. Cosmochim. Ac., 47, 1139–1150, 1983.
Hartman, W. K. and Davis, D. R.: Satellite-sized planetesimals and lunar origin, Icarus, 24, 504–515, 1975.
Hauri, E. H.: SIMS analysis of volatiles in silicate glasses, 2: isotopes and abundances in Hawaiian melt inclusions, Chem. Geol., 183, 115–141, 2002.
Hauri, E. H., Weinreich, T., Saal, A. E., Rutherford, M. J., and Van Orman, J. A.: High pre-eruptive water contents preserved in lunar melt inclusions, Science, 333, 213–215, 2011.
Hauri, E. H., Saal, A. E., Rutherford, M. J., and Van Orman, J. A.: Water in the moon's interior: truth and consequences, Earth Planet. Sc. Lett., 409, 252–264, 2015.
Heiken, G. H., Vaniman, D. T., and French, B. M. (Eds.): Lunar Sourcebook: A User's Guide to the Moon, Cambridge University Press, 1991.
Hirschmann, M. M.: Constraints on the early delivery and fractionation of Earth's major volatiles from C/H, C/N, and C/S ratios, Am. Mineral., 101, 540–552, 2016.
Hollister, L. S. and Crawford, M. L.: Melt immiscibility in Apollo 15 KREEP: origin of Fe-rich mare basalts, Proc. Lunar Sci. Conf. 8th, 2419–2432, 1977.
Hu, S., He, H. C., Ji, J. L., Lin, Y. T., Hui, H. J., Anand, M., Tartese, R., Yan, Y. H., Hao, J. L., Li, R. Y., Gu, L. X., Guo, Q., He, H. Y., and Ouyang, Z. Y.: A dry lunar mantle reservoir for young mare basalts of Change-5, Nature, 600, 49–53, 2021.
Jakobsen, J. K., Veksler, I. V., Tegner, C., and Brooks, C. K.: Immiscible iron- and silica-rich melts in basalt petrogenesis documented in the Skaergaard intrusion, Geology, 33, 885–888, 2005.
Jenner, F. E. and Arevalo, R. D.: Major and trace element analysis of natural and experimental igneous systems using LA-ICP-MS, Elements, 12, 311–316, 2016.
Johnson, M. C., Anderson Jr., A. T., and Rutherford, M. J.: Pre-eruptive volatile contents of magmas, Rev. Mineral., 30, 281–330, 1994.
Jolliff, B. L.: Fragments of quartz monzodiorite and felsite in Apollo 14 soil particles, Proc. Lunar Planet. Sci. Conf., 21, 101–118, 1991.
Jolliff, B. L., Floss, C., McCallum, I. S., and Schwartz, J. M.: Geochemistry, petrology, and cooling history of 14161,7373: a plutonic lunar sample with textural evidence of granitic-fraction separation by silicate-liquid immiscibility, Am. Mineral., 84, 821–837, 1999.
Koleszar, A. M., Saal, A. E., Hauri, E. H., Nagle, A. N., Liang, Y., and Kurz, M. D.: The volatile contents of the Galapagos plume; evidence for H2O and F open system behavior in melt inclusions, Earth Planet. Sc. Lett., 287, 442–452, 2009.
Kress, V. C. and Ghiorso, M. S.: Thermodynamic modeling of post-entrapment crystallization in igneous phases, J. Volcanol. Geotherm. Res., 137, 247–260, 2004.
Kyser, T. K., Lesher, C. E., and Walker, D.: The effects of liquid immiscibility and thermal diffusion on oxygen isotopes in silicate liquids, Contrib. Mineral. Petrol., 133, 373–381, 1998.
LAPST, L. A. P. S. T.: Horizons and Opportunities in Lunar Sample Science, Lunar and Planetary Institute, LPI Technical Report 85-04, 41 pp., https://www.lpi.usra.edu/lunar/strategies/sci_obj/tr85_04.pdf (last access: 24 January 2024), 1985.
Lin, Y., Tronche, E. J., Steenstra, E. S., and van Westrenen, W.: Evidence for an early wet Moon from experimental crystallization of the lunar magma ocean, Nat. Geosci., 10, 14–18, 2017a.
Lin, Y., Tronche, E. J., Steenstra, E. S., and van Westrenen, W.: Experimental constraints on the solidification of a nominally dry lunar magma ocean, Earth Planet. Sc. Lett., 471, 104–116, 2017b.
Lodders, K.: Solar system abundances and condensation temperatures of the elements, Astrophys. J., 591, 1220–1247, 2003.
Lowenstern, J. B.: Applications of silicate melt inclusions to the study of magmatic volatiles, Mineral. Assoc. Can. Short Course, 23, 71–99, 1995.
Lu, F., Anderson, A. T., and Davis, A. M.: Diffusional gradients at the crystal/melt interface and their effect on the composition of melt inclusions, J. Geol., 103, 591–597, 1995.
Lunar Sample Prelliminary Examination Team: Preliminary examination of lunar samples from Apollo 11: a physical, chemical, mineralogical, and biological analysis of 22 kilograms of lunar rocks and fines, Science, 165, 1211–1227, 1969.
McDonough, W. F. and Sun, S.-S.: The composition of the Earth, Chem. Geol., 120, 223–253, 1995.
Michael, P. J.: The concentration, behavior and storage of H2O in the suboceanic upper mantle: implications for mantle metasomatism, Geochim. Cosmochim. Ac., 52, 555–566, 1988.
Michael, P. J.: Regionally distinctive sources of depleted MORB: evidence from trace elements and H2O, Earth Planet. Sc. Lett., 131, 301–320, 1995.
Nakajima, M. and Stevenson, D. J.: Inefficient volatile loss from the Moon-forming disk: reconciling the giant impact hypothesis and a wet Moon, Earth Planet. Sc. Lett., 487, 117–126, 2018.
Newcombe, M. E., Fabbrizio, A., Zhang, Y., Ma, C., Le Voyer, M., Guan, Y., Eiler, J. M., Saal, A. E., and Stolper, E. M.: Chemical zonation in olivine-hosted melt inclusions, Contrib. Mineral. Petrol., 168, 1030, https://doi.org/10.1007/s00410-014-1030-6, 2014.
Ni, P., Zhang, Y., and Guan, Y.: Volatile loss during homogenization of lunar melt inclusions, Earth Planet. Sc. Lett., 478, 214–224, 2017.
Ni, P., Zhang, Y., Chen, S., and Gagnon, J.: A melt inclusion study on volatile abundances in the lunar mantle, Geochim. Cosmochim. Ac., 249, 17–41, 2019.
Nikogosian, I. K. and Sobolev, A. V.: Ion-microprobe analysis of melt inclusions in olivine: experience in estimating the olivine-melt partition coefficients of trace elements, Geochem. Int., 35, 119–126, 1997.
O'Neill, H. S. C., Berry, A. J., and Mallmann, G.: The oxidation state of iron in Mid-Ocean_Ridge Basaltic (MORB) glasses: implications for their petrogenesis and oxygen fugacities, Earth Planet. Sc. Lett., 504, 152–162, 2018.
Pahlevan, K., Karato, S., and Fegley, B.: Speciation and dissolution of hydrogen in the proto-lunar disk, Earth Planet. Sc. Lett., 445, 104–113, 2016.
Papike, J. J., Ryder, G., and Shearer, C. K.: Lunar samples, Rev. Mineral. Geochem., 36, 5-1 to 5-234, 1998.
Pettke, T.: In situ Laser-Ablation-ICP-MS chemical analysis of melt inclusions and prospects for constraining subduction zone magmatism, Mineral. Assoc. Can. Short Course, 36, 51–80, 2006.
Pettke, T., Halter, W. E., Webster, J. D., Aigner-Torres, M., and Heinrich, C. A.: Accurate quantification of melt inclusion chemistry by LA-ICPMS: a comparison with EMP and SIMS and advantages and possible limitations of these methods, Lithos, 78, 333–361, 2004.
Qin, Z., Lu, F., and Anderson, A. T.: Diffusive reequilibration of melt and fluid inclusions, Am. Mineral., 77, 565–576, 1992.
Roedder, E.: Origin and significance of magmatic inclusions, Bull. Mineral., 102, 487–510, 1979a.
Roedder, E.: Melt inclusions in 75075 – the problem of anomalous low-K inclusions in ilmenite revisited, Lunar Planet. Sci. Conf. Abstr., 1033–1035, 1979b.
Roedder, E. and Weiblen, P. W.: Silicate liquid immiscibility in lunar magmas, evidenced by melt inclusions in lunar rocks, Science, 167, 641–644, 1970a.
Roedder, E. and Weiblen, P. W.: Lunar petrology of silicate melt inclusions, Apollo 11 rocks, Proc. Apollo 11 Lunar Sci. Conf., 801–837, 1970b.
Roedder, E. and Weiblen, P. W.: Petrology of silicate melt inclusions, Apollo 11 and Apollo 12 and terrestrial equivalents, Proc. 2nd Lunar Sci. Conf., 1, 507–528, 1971.
Roedder, E. and Weiblen, P. W.: Silicate melt inclusions and glasses in lunar soil fragments from the Luna 16 core sample, Earth Planet. Sc. Lett., 13, 272–285, 1972a.
Roedder, E. and Weiblen, P. W.: Petrographic features and petrologic significance of melt inclusions in Apollo 14 and 15 rocks, Proc. 3rd Lunar Sci. Conf., 1, 251–279, 1972b.
Roedder, E. and Weiblen, P. W.: Petrology of some lithic fragments from Luna 20, Geochim. Cosmochim. Ac., 37, 1031–1052, 1973.
Roedder, E. and Weiblen, P. W.: High-silica inclusions in olivine of Luna-24 samples, Geophys. Res. Lett., 4, 485–488, 1977a.
Roedder, E. and Weiblen, P. W.: Compositional variation in late-stage differentiates in mare lavas, as indicated by silicate melt inclusions, Proc. Lunar Sci. Conf. 8th, 1767–1783, 1977b.
Roedder, E. and Weiblen, P. W.: Differentiation trends in mare lavas, as indicated by silicate melt inclusions, Lunar Planet. Sci. Conf. Abstr., 813–815, 1977c.
Roedder, E. and Weiblen, P. W.: Significance of melt inclusions in olivine of Luna 24 samples, Lunar Planet. Sci. Conf. Abstr., 970–972, 1978.
Roeder, P. L. and Emslie, R. F.: Olivine-liquid equilibrium, Contrib. Mineral. Petrol., 29, 275–289, 1970.
Rosenthal, A., Hauri, E. H., and Hirschmann, M. M.: Experimental determination of C, F, and H partitioning between mantle minerals and carbonated basalt, , systematics of partial melting and the CO2 contents of basaltic source regions, Earth Planet. Sc. Lett., 412, 77–87, 2015.
Saal, A. E. and Hauri, E. H.: Large sulfur isotope fractionation in lunar volcanic glasses reveals the magmatic differentiation and degassing of the Moon, Sci. Adv., 7, eabe4641, https://doi.org/10.1126/sciadv.abe4641, 2021.
Saal, A. E., Hauri, E. H., Langmuir, C. H., and Perfit, M. R.: Vapor undersaturation in primitive mid-ocean-ridge basalt and the volatile content of Earth's upper mantle, Nature, 419, 451–455, 2002.
Saal, A. E., Hauri, E. H., Cascio, M. L., Van Orman, J. A., Rutherford, M. J., and Cooper, R. F.: Volatile content of lunar volcanic glasses and the presence of water in the Moon's interior, Nature, 454, 192–196, 2008.
Saal, A. E., Hauri, E., Van Orman, J. A., and Rutherford, M.: Hydrogen isotopes in lunar volcanic glasses and melt inclusions reveal a carbonaceous chondritic heritage, Science, 340, 1317–1320, 2013.
Salters, V. J. and Stracke, A.: Composition of the depleted mantle, Geochem. Geophys. Geosys., 5, Q05004, https://doi.org/10.1029/2003GC000597, 2004.
Sato, M.: Electrochemical measurements and control of oxygen fugacity and other gaseous fugacities with solid electrolyte sensors, in: Research Techniques for High Pressure and High Temperature, edited by: Ulmer, G. C., Springer-Verlag, New York, 43–99, 1971.
Sato, M., Hickling, N. L., and McLane, J. E.: Oxygen fugacity values of Apollo 12, 14, and 15 lunar samples and reduced state of lunar magmas, Proc. 4th Lunar Sci. Conf. (Suppl. Geochim. Cosmochim. Acta), 1, 1061–1079, 1973.
Sharp, Z. D.: Nebular ingassing as a source of volatiles to the terrestrial planets, Chem. Geol., 448, 137–150, 2017.
Shimizu, K., Saal, A. E., Myers, C. E., Nable, A. N., Hauri, E. H., Forsyth, D. W., Kammenetsky, V. S., and Niu, Y.: Two-component mantle melting-mixing model for the generation of mid-ocean ridge basalts: implications for the volatile content of the Pacific upper mantle, Geochim. Cosmochim. Ac., 176, 44–80, 2016.
Sides, I., Edmonds, M., Maclennan, J., Houghton, B. F., Swanson, D. A., and Steele-MacInnis, M. J.: Magma mixing and high fountaining during the 1959 Kilauea Iki eruption, Hawaii, Earth Planet. Sc. Lett., 400, 102–112, 2014.
Siegler, M. A., Feng, J., Lehman-Franco, K., Andrews-Hanna, J. C., Economos, R. C., St. Clair, M., Million, C., Head, J. W., Glotch, T. D., and White, M. N.: Remote detection of a lunar granitic batholith at Compton-Belkovich, Nature, 620, 116–121, 2023.
Singer, J. A., Greenwood, J. P., Itoh, S., Sakamoto, N., and Yurimoto, H.: Evidence for the solar wind in lunar magmas: a study of slowly cooled samples of the Apollo 12 olivine basalt suite, Geochem. J., 51, 95–104, 2017.
Stephant, A., Anand, M., Tartese, R., Zhao, X., Degli-Alessandrini, G., and Franchi, L. A.: The hydrogen isotopic composition of lunar melt inclusions: an interplay of complex magmatic and secondary processes, Geochim. Cosmochim. Ac., 284, 196–221, 2020.
Stephant, A., Anand, M., Zhao, X., Chan, Q. H. S., Bonifacie, M., and Franchi, I. A.: The chlorine isotopic composition of the Moon: insights from melt inclusions, Earth Planet. Sc. Lett., 523, 115715, https://doi.org/10.1016/j.epsl.2019.115715, 2019.
Stolper, E. and Holloway, J. R.: Experimental determination of the solubility of carbon dioxide in molten basalt at low pressure, Earth Planet. Sc. Lett., 87, 397–408, 1988.
Su, X., Zhang, Y., Liu, Y., and Holder, R. M.: Outgassing and in-gassing of Na, K and Cu in lunar 74220 orange glass beads, Earth Planet. Sc. Lett., 602, 117924, https://doi.org/10.1016/j.epsl.2022.117924, 2023.
Sun, S. and McDonough, W. F.: Chemical and isotopic systematics of oceanic basalts: implications for mantle composition and processes, in: Magmatism in the Ocean Basins, edited by: Sauders, A. D. and Norry, M. J., Geological Society Special Publication, 313–345, https://doi.org/10.1144/GSL.SP.1989.042.01.19, 1989.
Taylor, G. J., Warner, R. D., Keil, K., Ma, M. S., and Schmidt, R. A.: Silicate liquid immiscibility, evolved lunar rocks and the formation of KREEP, Proc. Conf. Lunar Highlands Crust, 339–352, 1980.
Taylor, S. R., Pieters, C. M., and MacPherson, G. J.: Earth-Moon system, planetary science, and lessons learned, Rev. Mineral. Geochem., 60, 657–704, 2006.
Thomas, J. B., Bodnar, R. J., Shimizu, N., and Sinha, A. K.: Determination of zircon/melt trace element partition coefficients from SIMS analysis of melt inclusions in zircon, Geochim. Cosmochim. Ac., 66, 2887–2901, 2002.
Usselman, T. M., Lofgren, G. E., Donaldson, C. H., and Williams, R. J.: Experimentally reproduced textures and mineral chemistries of high-titanium mare basalts, Proc. Lunar Sci. Conf. 6th, 997–1020, 1975.
Wadhwa, M.: Redox conditions on small bodies, the Moon and Mars, Rev. Mineral. Geochem., 68, 493–510, 2008.
Walker, D., Longhi, J., and Hays, J. F.: Experimental petrology and origin of Fra Mauro rocks and soil, Geochim. Cosmochim. Ac., 1, 797–817, 1972.
Wallace, P. J., Kamenetsky, V. S., and Cervantes, P.: Melt inclusion CO2 contents, pressures of olivine crystallization, and the problem of shrinkage bubbles, Am. Mineral., 100, 787–794, 2015.
Wanke, H., Palme, H., baddenhausen, H., Dreibus, G., Jagoutz, E., Kruse, H., Palme, C., Spettel, B., Teschke, F., and Thacker, R.: New data on the chemistry of lunar samples: primary matter in the lunar highlands and the bulk composition of the Moon, Proc. Lunar Sci. Conf. 6th, 1313–1340, 1975.
Wanke, H., Palme, H., Kruse, H., baddenhausen, H., Cendales, G., Dreibus, G., Hofmeister, H., Jagoutz, E., Palme, C., Spettel, B., and Thacker, R.: Chemistry of lunar highland rocks: a refined evaluation of the composition of the primary matter, Proc. Lunar Sci. Conf. 7th, 3479–3499, 1976.
Wasserburg, G. J., MacDonald, G. J. F., Hoyle, F., and Fowler, W. A.: Relative contributions of uranium, thorium and potassium to heat production in the Earth, Science, 143, 465–467, 1964.
Weiblen, P. W.: Examination of the liquid line of descent of mare basalts in light of data from melt inclusions in olivine, Proc. Lunar Sci. Conf. 8th, 1751–1765, 1977.
Weiblen, P. W. and Roedder, E.: Petrology of melt inclusions in Apollo samples 15598 and 62295, and of clasts in 67915 and several lunar soils, Proc. 4th Lunar Sci. Conf., 1, 681–703, 1973.
Weiblen, P. W. and Roedder, E.: Compositional interrelationships of mare basalts from bulk chemical and melt inclusion studies, Proc. Lunar Sci. Conf. 7th, 1449–1466, 1976a.
Weiblen, P. W. and Roedder, E.: Comparative compositional studies of silicate melt inclusion, bulk chemical, and experimental data on mare basalts, Lunar Planet. Sci. Conf. Abstr., 927–929, 1976b.
Wetzel, D. T., Hauri, E. H., Saal, A. E., and Rutherford, M. J.: Carbon content and degassing history of the lunar volcanic glasses, Nat. Geosci., 8, 755–758, 2015.
Wood, B. J., Smythe, D. J., and Harrison, T.: The condensation temperatures of the elements: a reappraisal, Am. Mineral., 104, 844–856, 2019.
Workman, R. K., Hauri, E. H., Hart, S. R., Wang, J., and Blusztajin, J.: Volatile and trace elements in basaltic glass from Samoa: implications for water distribution in the mantle, Earth Planet. Sc. Lett., 241, 932–951, 2006.
Xirouchakis, D., Hirschmann, M. M., and Simpson, J. A.: The effect of titanium on the silica content and on mineral-liquid partitioning of mantle-equilibrated melts, Geochim. Cosmochim. Ac., 65, 2201–2217, 2001.
Zeng, X., Joy, K. H., Li, S., Lin, Y., Wang, N., Li, X., Li, Y., Hao, J., Liu, J., and Wang, S.: Oldest immiscible silica-rich melt on the Moon recorded in a ∼ 4.38-Ga zircon, Geophys. Res. Lett., 47, e2019GL085997, https://doi.org/10.1029/2019GL085997, 2020.
Zhang, Y.: Quantification of the elemental incompatibility sequence, and composition of the “superchondritic” mantle, Chem. Geol., 369, 12–21, 2014.
Zhang, Y.: H2O and other volatiles in the Moon, 50 years and on, ACS Earth Space Chem., 4, 1480–1499, 2020.
- Abstract
- Introduction
- Methods and possible complexities
- Early studies of MIs in lunar rocks
- More recent (2011–now) studies of MIs in lunar rocks
- Summary
- Future perspectives
- Data availability
- Competing interests
- Disclaimer
- Special issue statement
- Acknowledgements
- Financial support
- Review statement
- References
- Abstract
- Introduction
- Methods and possible complexities
- Early studies of MIs in lunar rocks
- More recent (2011–now) studies of MIs in lunar rocks
- Summary
- Future perspectives
- Data availability
- Competing interests
- Disclaimer
- Special issue statement
- Acknowledgements
- Financial support
- Review statement
- References