the Creative Commons Attribution 4.0 License.
the Creative Commons Attribution 4.0 License.
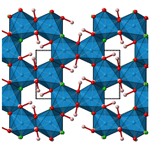
Nannoniite, Al2(OH)5F, a new mineral from the Cetine di Cotorniano mine (Tuscany, Italy)
Cristian Biagioni
Enrico Mugnaioli
Sofia Lorenzon
Daniela Mauro
Silvia Musetti
Jiří Sejkora
Donato Belmonte
Nicola Demitri
Zdeněk Dolníček
The new mineral nannoniite, Al2(OH)5F (Z=4), has been discovered in the Cetine di Cotorniano mine, Chiusdino, Siena, Tuscany, Italy. It occurs as spherical aggregates formed by micrometre-sized crystals, colourless to white in colour, with a white streak and a vitreous to earthy lustre. Fluorescence is bluish-yellow and yellowish-white under short- and longwave UV radiation, respectively. Electron microprobe analysis gave (in wt %) SO3 0.49, Al2O3 63.97, MgO 0.51, CaO 0.22, K2O 0.07, F 11.72, H2O(calc) 28.54, and −O = F −4.94, with a total of 100.58. Nannoniite is monoclinic, with space group and with a=8.688(3), b=5.024(2), c=9.734(4) Å, β=90.77(2)°, and V=424.9(3) Å3. The crystal structure was solved using three-dimensional electron diffraction and refined to R(obs)=0.1524 for 2141 unique reflections with I>3σ(I). Nannoniite is homeotypic with gibbsite. In type material, nannoniite is associated with quartz, baryte, gypsum, and alunite in vugs of a silicified limestone. Its origin is probably related to the late-stage circulation of (Al,F)-rich fluids within the Sb ore deposit formerly exploited at the Cetine di Cotorniano mine.
- Article
(5698 KB) - Full-text XML
-
Supplement
(456 KB) - BibTeX
- EndNote
The Cetine di Cotorniano mine is one of the most interesting mineralogical localities in Tuscany (Italy), being the type locality of some mineral species: batoniite, brizziite, cetineite, onoratoite, and rosenbergite (Belluomini et al., 1968; Sabelli and Vezzalini, 1987; Olmi et al., 1993; Olmi and Sabelli, 1994; Mauro et al., 2023). The first new mineral found at this locality was onoratoite, Sb8O11Cl2, whose origin is related to the alteration of stibnite. During the intense collecting activity performed by mineral amateurs from the 1970s, other species were identified. Brizziite, NaSbO3, and cetineite, NaK5Sb14S6O18(H2O)6, were found in vugs of metallurgical slags, whereas the recently described mineral batoniite, [Al8(OH)14(H2O)18](SO4)5⋅ 5H2O, was identified in sulfate assemblages occurring in the lower mining level of the Cetine di Cotorniano mine. Finally, rosenbergite, AlF[F0.5(H2O)0.5]4⋅ H2O, was discovered in a small exploitation void currently known among mineral collectors as “Stanza Santoni”, after the mineral collector Valerio Santoni (1934–2002), who usually looked for minerals in this area (Amici Mineralogisti Fiorentini, 2002). The interest for this area is mainly related to the presence of some rare fluorides. Indeed, in addition to rosenbergite, well-crystallized specimens of elpasolite, K2NaAlF6, and hydrokenoralstonite, □2Al2F6(H2O), could be collected there (Menchetti and Batoni, 2015).
During the routine analyses for the identification of mineral specimens from the Cetine di Cotorniano mine provided by several mineral collectors (Tiberio Bardi, Massimo Batoni, and Paolo Gianinoni), a mineral species having an X-ray powder diffraction pattern similar, but not identical, to that of gibbsite was identified. Later qualitative energy-dispersive X-ray spectroscopic (EDS) chemical analysis showed the ubiquitous occurrence of F in these samples, leading to the identification of the studied material as the unnamed mineral, reported as UM1990-28-OHF:Al (Smith and Nickel, 2007), described by Jambor et al. (1990) from the Francon quarry, Montreal, Quebec, Canada. The chemical and structural study performed on two further specimens provided by Massimo Batoni allowed the full characterization of this species.
The mineral, its name, and its symbol (Nnn) were approved by the Commission on New Minerals, Nomenclature and Classification of the International Mineralogical Association (CNMNC-IMA) under voting number 2024-010. The name honours Roberto Nannoni (1943–2022) for his contribution to the knowledge of Tuscan mineralogy. Roberto graduated in geological sciences at the University of Pisa in 1967. After graduating, he became a teacher of chemistry, biology, and earth sciences at secondary schools. He was a collaborator of the Museo Provinciale di Storia Naturale of Leghorn from 1972 to 1998, and, during the 1980s, he was honorary curator of the mineralogical collection of that museum. He published some books about regional mineralogy and several papers in some Italian journals (e.g. “Atti della Società Toscana di Scienze Naturali”, “Quaderni del Museo di Storia Naturale di Livorno”, and “Rivista Mineralogica Italiana”). The type material of nannoniite is deposited in the mineralogical collection of the Museo di Storia Naturale, University of Pisa, Via Roma 79, Calci (Pisa), under catalogue numbers 20071 (holotype) and 20072 (co-type).
This paper describes the new mineral species nannoniite and discusses its occurrence and crystal chemistry.
Nannoniite was identified in two samples collected at the Cetine di Cotorniano mine (latitude 43°13′ N, longitude 11°09′ E), Chiusdino, province of Siena, Tuscany, Italy. This mine was active between 1878 and 1948, exploiting an Sb ore deposit whose origin is related to the hydrothermal activity having widely developed in southern Tuscany since the Late Miocene and associated with the geothermal anomaly induced by the magmatic rocks belonging to the Tuscan Magmatic Province (e.g. Lattanzi, 1999; Dini, 2003; Sillitoe and Brogi, 2021). The mineralization consists of jasperoids and vuggy silica masses replacing host rocks (“Calcare Cavernoso” formation of Upper Triassic age) at the contact with the overlying argillic formations belonging to the Liguride Nappe. Ore minerals are mainly represented by stibnite, whereas iron sulfides (pyrite, marcasite) are accessory phases.
In type material, nannoniite occurs as (hemi-)spherical aggregates, up to 1 mm in diameter, formed by spindle-like crystals (Fig. 1a) or tabular individuals (Fig. 1b). Nannoniite is colourless to white in colour, with a white streak and a vitreous to earthy lustre. The mineral shows a bluish-white fluorescence under shortwave UV radiation (λ=254 nm) and a yellowish-white fluorescence under longwave UV radiation (λ=350 nm). Mohs hardness was not determined owing to the small crystal size; in analogy with gibbsite, it may be close to 2–3. Nannoniite is brittle and shows a perfect {001} cleavage. Density was not measured. Calculated density, based on the ideal formula and unit-cell parameters, is 2.492 g cm−3. The mean refractive index, calculated according to the Gladstone–Dale relationship (Mandarino, 1979, 1981), is 1.564.
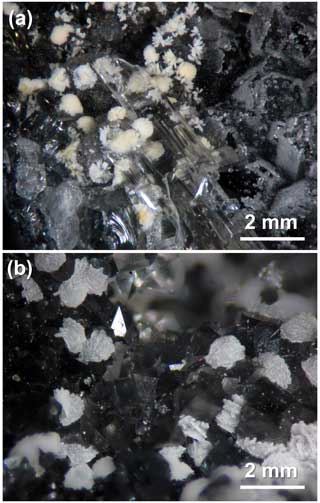
Figure 1Nannoniite from the Cetine di Cotorniano mine, Chiusdino, province of Siena, Tuscany, Italy. (a) Aggregates of whitish spindle-like crystals, up to 0.5 mm in diameter, associated with colourless gypsum and small grains of alunite (on right) on quartz. (b) Rosette-like aggregates of tabular crystals, up to 1 mm in diameter, on quartz. Holotype and co-type material. Collection of Museo di Storia Naturale of the University of Pisa, catalogue numbers 20071 (a) and 20072 (b). Photo Cristian Biagioni.
In type material, nannoniite is associated with quartz, baryte, gypsum, and alunite in vugs of silicified limestone. The specimens were collected in the small exploitation void known as “Stanza Santoni”. As written above, in this area, several fluorides have been identified, e.g. elpasolite, fluorite, gearksutite, hydrokenoralstonite, and rosenbergite (Menchetti and Batoni, 2015), as well as the new mineral species dacostaite (IMA 2024-015; Biagioni et al., 2024). Nannoniite is a further addition to this list.
3.1 Raman spectroscopy
Micro-Raman spectra were collected on a sample of nannoniite in the range of 100–4000 cm−1 using a HORIBA Jobin Yvon XploRA Plus apparatus mounted on an Olympus BX41 microscope and equipped with a motorized x–y stage and a 50× objective (Dipartimento di Scienze della Terra, Università di Pisa, Italy). The Raman signal was excited by a green 532 nm solid-state laser and detected by a CCD detector. The minimum lateral and depth resolution was set to a few micrometres. The system was calibrated using the 520.6 cm−1 Raman band of silicon before each experimental session. Spectra were collected through multiple acquisitions (three) with single counting times of 80 s. Backscattered radiation was analysed with a 1200 gr mm−1 grating monochromator. The possible thermal damage of the measured points was excluded through the visual inspection of the excited surface after measurement by checking for the decay of the spectral lines at the start of excitation and for thermal downshift of Raman lines.
3.2 Chemical data
Quantitative chemical analyses were carried out using a Cameca SX 100 electron microprobe (wavelength-dispersive X-ray spectroscopy (WDS) mode, 15 kV, 5 nA, 20 µm beam diameter) on a polished surface at the National Museum, Prague, Czech Republic. Standards (element, emission line) were celestine (SKα), Al2O3 (AlKα), diopside (MgKα), fluorapatite (CaKα), sanidine (KKα), and LiF (FKα). The contents of other analysed elements (i.e. Na, P, Si, Cl, Fe, Cu, Zn, and As) are below detection limits. Matrix correction by PAP procedure (Pouchou and Pichoir, 1985) was applied to the data. The results of chemical analyses (average of nine spot analyses) are given in Table 1. Because insufficient pure material is available for a direct determination of H2O, the content of this latter chemical constituent has been calculated based upon the known stoichiometry from structure analysis.
Table 2X-ray powder diffraction data (d in Å) for nannoniite compared with those of UM1990-28-OHF:Al (Jambor et al., 1990).
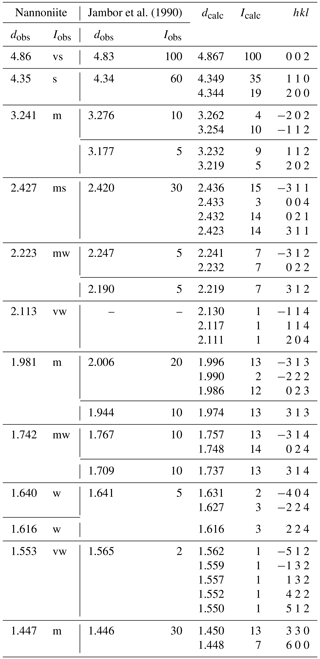
Icalc and dcalc were obtained using PowderCell 2.4 (Kraus and Nolze, 1996) on the basis of the structural model of nannoniite given in Table 4. Only calculated reflections with Icalc>2 (if not observed) are given. Iobs were visually estimated: vs is very strong, s is strong, ms is medium-strong, m is medium, mw is medium-weak, w is weak, and vw is very weak.
3.3 X-ray crystallography
Owing to the small amount of pure material, X-ray powder diffraction data of nannoniite were collected using a Bruker D8 Venture single-crystal diffractometer equipped with a Photon III CCD area detector and microfocus CuKα radiation (Centro per l'Integrazione della Strumentazione scientifica dell'Università di Pisa, CISUP, Pisa, Italy), simulating a Gandolfi-like geometry. Observed X-ray diffraction lines are reported in Table 2, along with the calculated pattern based on the structural model discussed below and that given by Jambor et al. (1990) for UM1990-28-OHF:Al. Unit-cell parameters were refined using the software Celref (Laugier and Bochu, 1999) and are a=8.680(3), b=5.018(4), c=9.711(4) Å, β=90.26(9)°, and V=423.0(4) Å3, with space group . These cell settings, with the shortest a and c vectors, and space group were preferred to the cell settings corresponding to the conventional space group to be consistent with those of gibbsite (Saalfeld and Wedde, 1974).
Only one grain, µm in size, was found suitable for single-crystal X-ray diffraction study using a Bruker D8 Venture single-crystal diffractometer with a Photon III CCD area detector and microfocus MoKα radiation (CISUP, Pisa, Italy). Unit-cell parameters are based on the refinement of the XYZ centroids of 122 reflections above 20σ(I) with . They are a=8.672(7), b=5.009(4), c=9.673(8) Å, β=90.50(3)°, and V=420.2(6) Å3. Systematic absences suggested the space group . However, reflections were very weak, and notwithstanding the very long exposure (300 s per frame) the number of observed reflections was low, i.e. only 159 reflections with Fo>4σ(Fo). Further attempts to improve the data quality were performed using synchrotron radiation at the Elettra Sincrotrone (Trieste, Italy) on the same grain used for single-crystal X-ray diffraction. Unfortunately, this experiment failed due to sample decay.
3.4 Electron crystallography
Owing to the very small size of the available single crystals, nannoniite was examined through three-dimensional electron diffraction (3DED) (Kolb et al., 2007; Mugnaioli et al., 2009; Mugnaioli and Gemmi, 2018; Gemmi et al., 2019) using a JEOL JEM-F200 multipurpose transmission electron microscope (TEM) operating at 200 kV and equipped with a Schottky field emission gun (FEG) source and an ASI CheeTah hybrid single-electron detector (516×516 pixels, 24 bit) (CISUP, Pisa, Italy). The unit-cell parameters, determined using PETS2 (Palatinus et al., 2019), are a=8.688(3), b=5.024(2), c=9.734(4) Å, β=90.77(2)°, and V=424.9(3) Å3. Systematic absences agree with the space group . The discrepancy between X-ray and electron diffraction data is interpreted as being due to the weakness of the former data, simulating a C-centred lattice.
The 3DED dataset was acquired in scanning transmission electron microscopy (STEM) mode with a pseudo-parallel illumination, obtained through a 10 µm condenser aperture and a designated lens configuration. Each frame of the dataset was collected with an angle step of ∼1° with a precessing beam to improve reflection integration and reduce excitation error. The beam precession was controlled by a NanoMEGAS Topspin digital controller, and the precession semi-angle was set at 1°. A total of 121 frames were collected to resolve the structure. The camera length was set at 250 mm with a pixel resolution of 0.00583 Å. The structure of nannoniite was solved ab initio using SIR2019 (Burla et al., 2015) in the space group using standard direct methods and neglecting any dynamical effect. The maxima in the potential map correspond to two cations (Al atoms), six anions (O F atoms), and three H atoms. These latter atoms are the out-of-plane H, hosted at sites H(3), H(4), and H(5).
The structure refinement was performed using JANA2020 (Petříček et al., 2014), considering dynamical scattering and using the empirical chemical formula obtained through electron microprobe analysis. A total of 104 of 121 zones were used for the final refinement, excluding the zones collected at high tilt angle and high thickness. To determine the O F occupancy in anion sites, the same O F ratio, as determined by WDS analyses, was set at the three anion sites not bonded to the H atoms determined in the ab initio solution. Subsequently, the occupancy of O F was allowed to freely refine. At the end of this refinement cycle, one of the anion sites, namely the O(2) site, was almost completely occupied by F. The preference of F for the O(2) [= F(2)] site is also supported by the following evidence:
-
Adding the two lacking H atoms, their positions refine quite well with the expected ones for a gibbsite-like mineral (namely the atoms hosted at the H(1) and H(6) site).
-
Refining the O F occupancy in all six anion sites (not only in the three sites where H was not resolved in the ab initio model), F still tends to occupy the O(2) position.
-
Adding the H atoms associated with all anion sites in the structure, the H atoms bonded to O(2) does not refine to a realistic position; conversely, the H(1) and H(6) sites can be refined even if some restraints should be applied in the final stages of the refinement in order to give a sound geometry of the H bonds.
Table 3Crystal and experimental data for nannoniite.
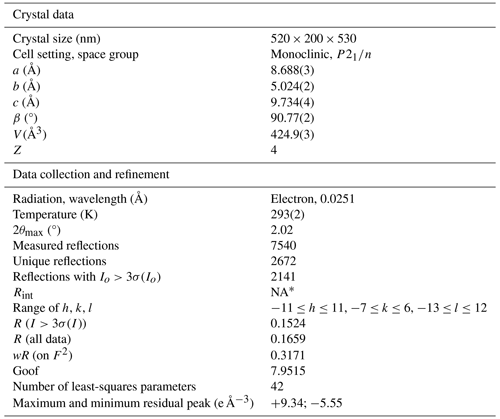
* NA – not available. For dynamical structure refinement against electron diffraction data, each reflection is considered individually, and therefore Rint parameter has no significance.
Table 4Sites, site occupancy (s.o.), fractional atom coordinates, and isotropic displacement parameters (in Å2) for nannoniite.
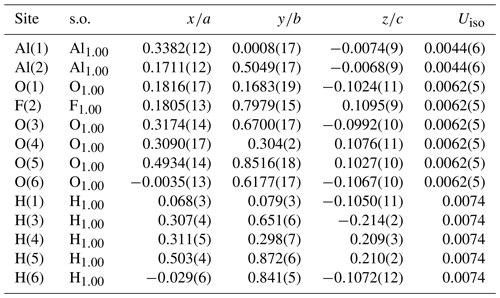
In the final refinement cycles, the same isotropic displacement parameters for all O F atoms and the two Al atoms were imposed. Moreover, O–H distances were restrained to 1.00(1) Å and the positions of the H(1) and H(6) sites were restrained in order to achieve a physically sound configuration of the H bonds. The refinement converged to a final R(obs)=0.1524 for 2141 unique reflections with I>3σ(I) and 42 refined parameters. Details of the data collection and crystal structure refinement are given in Table 3. Atom coordinates and displacement parameters are reported in Table 4, whereas Table 5 gives selected bond distances. A bond-valence calculation, shown in Table 6, was performed using the bond parameters of Brese and O'Keeffe (1991). Table 7 gives details of the possible O–H bonds.
4.1 Raman spectrum of nannoniite
The main bands observed in the Raman spectrum of nannoniite in the region between 100 and 1200 cm−1 are (in cm−1; Fig. 2a) 147, 187, 251, 303, 371, 446, 561 (with a shoulder at 534), 697, 900, 976, and 1021. Bands at 900, 976, and 1021 cm−1 could be interpreted as hydroxyl deformation modes δ(OH), in agreement with previous authors (e.g. Ruan et al., 2001), who reported the occurrence of δ(OH) modes in gibbsite at 916, 980, 1019, and 1051 cm−1. The strong band at 561 cm−1, with a shoulder towards lower wavenumbers at 534 cm−1, could be due, in agreement with previous studies (e.g. Frost et al., 1999; Ruan et al., 2001), to Al–O–Al deformation, whereas the weak band at 697 cm−1 could be related to hydroxyl translation modes (Ruan et al., 2001). Another strong band occurs at 251 cm−1. This band, as well as the weaker band at 303 cm−1, could be assigned to Al–O–Al stretching modes, whereas the band at 446 cm−1 may be due to the Al–O–Al bending (Frost et al., 1999; Ruan et al., 2001). Bands at wavenumbers lower than 200 cm−1 are probably related to lattice vibrations.
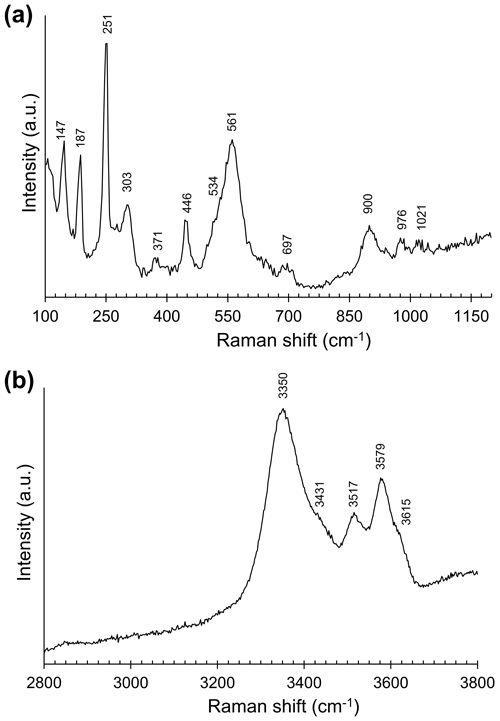
Figure 2Raman spectrum of nannoniite in the range 100–1200 (a) and 2800–3800 cm−1 (b). The abbreviation “a.u.” signifies arbitrary unit.
In the O–H stretching region (Fig. 2b), nannoniite shows three main bands at 3350, 3517, and 3579 cm−1 with shoulders at 3431 and 3615 cm−1. These positions can be compared with those reported by previous authors for gibbsite, e.g. Huneke et al. (1980) (3361, 3431, 3520, and 3615 cm−1), Rodgers (1993) (3365, 3425, 3525, and 3618 cm−1), and Ruan et al. (2001) (3364, 3433, 3522, and 3617 cm−1). Balan et al. (2006, 2008) suggested that in-plane OH groups show higher Raman shifts than out-plane OH groups in both bayerite and gibbsite. However, these authors admitted that a straightforward interpretation of the O–H stretching modes could be difficult, and this same statement could probably also be applied to nannoniite.
4.2 Chemical formula of nannoniite
On the basis of (OH + F) =6 atoms per formula unit, the empirical formula of nannoniite is (Al1.99Mg0.02)Σ2.01(SO4)0.01(OH)5.02F0.98. The simplified formula of this mineral is (Al,Mg)2(OH)5(F,OH). The ideal one is Al2(OH)5F, corresponding to (in wt %) Al2O3 64.53, F 12.02, H2O 28.51, and −O = F −5.06, with a sum of 100.00.
As shown in Table 1, in addition to Al and F, some other minor chemical elements were detected during WDS chemical analyses. Minor amounts of Ca, K, and S (possibly as SO4 groups) are probably due to the intimate association of nannoniite with both alunite and gypsum. Indeed, Raman spectroscopy (see above) and structure refinement (see below) do not support the occurrence of structural SO4 groups in nannoniite. Silicon, along with Mg and S, was detected through EDS analyses performed using a high-counting JEOL silicon drift detector (SDD) installed on the JEOL JEM-F200 Multipurpose TEM used for the collection of electron diffraction data. The EDS data were treated with a standardless thin foil Cliff–Lorimer approximation (Cliff and Lorimer, 1975) and gave the empirical formula Al2(OH)4.72F1.28, quite in agreement with WDS microprobe data. Silicon was also reported by Jambor et al. (1990) for UM1990-28-OHF:Al, and it was interpreted as being due to disseminated amorphous silica. This interpretation is probably applicable also to the material from the Cetine di Cotorniano mine, where opal has been reported in the same occurrence of nannoniite (Menchetti and Batoni, 2015). Probably the only minor element structurally bonded in nannoniite is Mg. This could be hosted according to the substitution rule 2Al 3Mg2+.
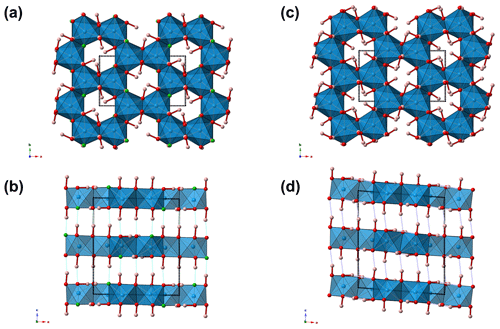
Figure 3Crystal structure of nannoniite as seen down c (a) and b (b). For the sake of comparison, the crystal structure of gibbsite is also shown (c, d). Symbols: blue polyhedra signify Al-centred octahedra. Circles: red denotes O atoms, green F atoms, and pink H atoms. Unit cells are shown as dotted lines.
4.3 Crystal structure of nannoniite
4.3.1 General features and cation coordination
The crystal structure of nannoniite is homeotypic with that of gibbsite (Fig. 3). It is a sheet structure formed by double layers of (OH F) atoms with Al cations occupying two-thirds of the octahedral interstices within the layers. The same ABBAABBA stacking of anions reported for gibbsite is shown by nannoniite. Hydrogen bonds occur between OH and F anions of successive double layers.
Two symmetry-independent Al-centred sites occur in nannoniite, as well as in homeotypic gibbsite. Aluminium–φ (φ= OH, F) distances range between 1.77 and 2.06 Å, whereas in gibbsite they are in the range of 1.83–1.95 Å (Saalfeld and Wedde, 1974). The differences between the longest and shortest distances at the octahedra around the Al(1) and Al(2) sites are 0.22 and 0.17 Å compared to 0.095 and 0.085 Å observed in gibbsite (Saalfeld and Wedde, 1974). The difference between the longest and shortest Al–φ distances in the Al-centred octahedra observed in nannoniite is similar to that reported by Megaw (1934) in her first determination of the monoclinic crystal structure of gibbsite, i.e. 0.25 and 0.34 Å for the two Al sites. The distortion observed in nannoniite is probably, at least partially, due to the relatively low quality of the electron diffraction data. Average bond distances are 1.91 and 1.88 Å for the Al(1) and Al(2) sites compared to 1.90 and 1.91 Å reported by Saalfeld and Wedde (1974) for gibbsite. Bond-valence sums are 2.95 and 3.11 valence units for Al(1) and Al(2), respectively.
An unusual feature shown by nannoniite is the long Al(1)–F(2) distance, which is the longest one at 2.06 Å. Indeed, using the bond parameters of Brese and O'Keeffe (1991), the calculated < Al–O > and < Al–F > bond distances should be 1.907 and 1.801 Å, with the Al–F distance that should be shorter than Al–O distances. This is what has been observed in some F-bearing phases, e.g. in topaz (< Al–F > = 1.801 Å; < Al–O > 1.902 Å; Gatta et al., 2006) and in khademite (Al–F = 1.732 Å, < Al–O > 1.906 Å; Mauro et al., 2020). In zharchikhite, the < Al–F > distance is slightly longer, i.e. 1.849 Å (Zubkova et al., 2024). In the crystal structure of nannoniite, every anion is bonded to two Al atoms, with the following average distances (in Å): O(1) 1.886, F(2) 1.960, O(3) 1.834, O(4) 1.912, O(5) 1.881, and O(6) 1.909. Whereas the O(1), O(4), O(5), and O(6) atoms show average distances agreeing with the ideal < Al–O > distance of 1.907 Å (considering the quality of the structural refinement), F(2) and O(3) significantly deviate from this average value. In particular, one could hypothesize that O(3) is the F-hosting site and F(2) could be an OH position. However, as discussed above, the crystal structure refinement suggests that fluorine is partitioned at F(2) and that a strong electron density maximum clearly indicates the occurrence of an out-of-plane H atom bonded to O(3). Consequently, the lengthening of the Al–F distance could occur due to uncertainties in the structural model or it may be real and related to the fact that F could be the acceptor of a relatively strong H bond from O(4), as well as of two other weaker H bonds from O(1) and O(6).
4.3.2 Hydrogen bonds
In nannoniite, O–H bonds can be distinguished between out-of-plane and in-plane, with reference to their position with respect to the sheets formed by Al-centred octahedra. As discussed above, the position of three out-of-plane H atoms was identified during the first stages of the crystal structure refinement. In contrast, some difficulties were encountered in the reliable detection of the position of the in-plane H atoms because electron density maps show weak and more diffuse peaks situated approximately within the O plane. Eventually, an ordered structural model was obtained, with the identification of two possible H positions corresponding to in-plane O–H bonds.
The three out-of-plane H bonds involve the H atoms bonded to O(3), O(4), and O(5). The D A distances for the O(3) O(1), O(4) F(2), and O(5) O(6) H bonds range between 2.75 and 2.91 Å compared to the O O distances given by Saalfeld and Wedde (1974) for gibbsite, i.e. 2.78, 2.83, and 2.89 Å. Bond angles vary between 169 and 178° (Table 7).
The D A distances of the in-plane H bonds are longer than those of the out-of-plane H bonds and display smaller D–H A angles. This could indicate a weak nature of these H bonds, as hypothesized by Clark et al. (1998) for doyleite, or it could be due to the uncertainty in the correct location of these two H bonds due, for instance, to rotational disorder of the Al(OH/F)3 layers. Indeed, some shortcomings in the correct identification of the in-plane H atoms bonded to O(1) and O(6) remain, and their geometry was fixed. However, some problems are still present, as, for instance, the H(1)–H(6) distance is only 1.5 Å, whereas in gibbsite the shortest H–H distance is 1.96 Å (Saalfeld and Wedde, 1974).
According to Ferraris and Franchini-Angela (1972) and Brown (1976), O O distances longer than 3.1–3.2 Å do not represent H bonds. Moreover, the D–H A angles are usually larger than 130° (Ferraris and Franchini-Angela, 1972; Pedersen, 1974; Brown, 1976). Consequently the O(1) O(6) and O(6) O(1) distances should not be considered as H bonds, as they show very long D A distances and small D–H A angles (less than 130°). The H bonds involving O(1) and O(6) as donors to F(2) are probably weak/very weak, as the D A distance is long and the bond angles are small, in particular for the O(1)–H(1) F(2) bond.
In agreement with Brown and Altermatt (1985), an estimation of the bond strengths of the H bonds occurring in nannoniite can be given (Table 7). Bond-valence sums, corrected for H bonds, agree with the monovalent nature of anions occurring in nannoniite (Table 6).
4.4 Comparison between nannoniite and related phases
Table 8 reports the currently known aluminium hydroxides. Among them, zharchikhite, AlF(OH)2 (Bolokhontseva et al., 1988; Zubkova et al., 2024), is the only one containing F as an essential chemical constituent, along with nannoniite. Its crystal structure is different, being related to α-PbO2. A mineral with the stoichiometry Al(OH)2F, similar but not identical to that of nannoniite, was also reported by Kasatkin et al. (2022), who interpreted it as a F analogue or a variety of nordstrandite.
Table 8Natural aluminium hydroxides.
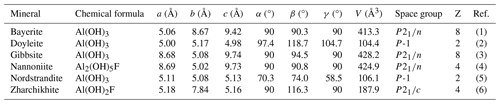
(1) Zigan et al. (1978). (2) Clark et al. (1998). (3) Saalfeld and Wedde (1974). (4) This work. (5) Bosmans (1970). (6) Zubkova et al. (2024).
4.4.1 Nannoniite vs. gibbsite
As stated above, nannoniite shows homeotypic relations with gibbsite. It is worth noting that the unit-cell volume of the latter is ∼428 Å3, larger than that of nannoniite (ΔV in the range +0.8–+1.9 %, on the basis of the unit-cell parameters measured using electron diffraction or single-crystal X-ray diffraction, respectively). This is in keeping with the larger effective ionic radius of the (OH) group, which is about 2.7 % larger than that of F in two-fold coordination (Shannon, 1976). Another meaningful difference between nannoniite and gibbsite is to be sought in the X-ray powder diffraction patterns (Fig. 4). As one can see, the two patterns are similar but not identical, with several notable differences. These are due to the values of their unit-cell parameters (Table 8). Indeed, even if the a, b, and c values are very similar, the β angle is distinctly different, being ∼91° in nannoniite and ∼94.5° in gibbsite. These different angular values are probably related to the distribution of H atoms (Fig. 3b and d). Such a different H configuration can also be observed in Fig. 5, where a single {001} layer of nannoniite is compared with a layer of gibbsite. The dioctahedral layers show pseudo-hexagonal cavities lined by anions that can be bonded to H atoms. Along a, rows of two different kinds of cavities can be distinguished. In nannoniite, type 1 cavities show four in-plane OH groups and two F atoms, i.e. O(1), F(2), and O(6) sites, whereas type 2 cavities are lined by six anions represented by six out-of-plane OH groups, i.e. the O(3), O(4), and O(5) sites. H atoms point alternatively up (U) and down (D), with the sequence UDUDUD. In gibbsite, the configuration is different. Type 1 cavities are lined by four in-plane OH groups (at O(1) and O(4) sites) and two out-of-plane OH groups (at the O(6) site), pointing U and D. Type 2 cavities show four out-of-plane (at the O(3) and O(5) sites) and two in-plane OH groups (at the O(2) site). Out-of-plane H atoms follow the sequence UUDD. This is an important structural feature clearly distinguishing nannoniite and gibbsite.
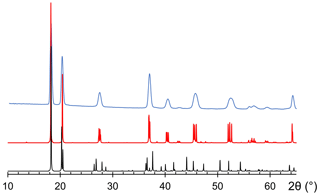
Figure 4Comparison between observed (light blue) and calculated (red) X-ray powder diffraction patterns for nannoniite. For the sake of comparison, the calculated X-ray powder diffraction pattern of gibbsite, based on the model given by Saalfeld and Wedde (1974), is shown (black).
Moreover, the (OH) F ratio in nannoniite is close to . This restricted chemical variability may suggest an ordering of F atoms in one of the anion positions. Structural study suggests that F is hosted in the type 1 cavities of nannoniite, at the F(2) site, and for this reason the endmember formula is given as Al2(OH)5F (Z=4).
4.4.2 Relations between nannoniite and UM1990-28-OHF:Al
Table 2 compares the X-ray powder diffraction pattern with that reported by Jambor et al. (1990) for UM1990-28-OHF:Al from the Francon quarry, Montreal, Canada. The two patterns are very similar and agree with the calculated pattern based on the structural model. Unit-cell parameters given by Jambor et al. (1990) are a=8.66, b=4.99, c=9.67 Å, β=92.12°, and V=417.6 Å3, to be compared to the values observed for nannoniite, i.e. a=8.69, b=5.02, c=9.73 Å, β=90.8°, and V=424.9 Å3. Jambor et al. (1990) reported several chemical analyses and recalculated three average compositions that showed variable Si contents as well as H2O groups. However, according to these authors, the Si content is due to disseminated amorphous silica, H2O groups are related to absorbed water, and the actual composition of the Francon mineral should be Al[(OH)1−xFx]3 (Z=8). According to their chemical analyses, , thus fitting with the formula of nannoniite, Al2(OH)5F (Z=4). The calculated density of nannoniite, i.e. 2.49 g cm−3, is comparable with the empirical and calculated density of UM1990-28-OHF:Al, i.e. 2.43 and 2.51 g cm−3 (Jambor et al., 1990). Moreover, nannoniite displays a bluish-white fluorescence under shortwave UV radiation (λ=254 nm) and a yellowish-white fluorescence under longwave UV radiation (λ=350 nm). These features compare well with the fluorescence observed by Jambor et al. (1990) for UM1990-28-OHF:Al, i.e. bluish white (short UV) and yellowish white (long UV). However, it should be remembered that gibbsite could also be fluorescent, as Robbins (1994) reported white and green fluorescence under longwave and shortwave UV radiation, respectively.
4.5 Nannoniite and other aluminium fluorides from the Cetine di Cotorniano mine
Nannoniite is relatively common in the cavities of silicified limestone of Stanza Santoni. Indeed, X-ray powder diffraction patterns collected on several specimens previously labelled as gibbsite revealed that the latter corresponds to nannoniite. The occurrence of gibbsite was first reported from the Cetine di Cotorniano mine by Brizzi et al. (1986), in association with gypsum and quartz. However, its physical properties and its occurrence fully agree with those of nannoniite (see, for instance, Menchetti and Batoni, 2015), and it is not unlikely that gibbsite was misidentified.
The mineral assemblage at Stanza Santoni is characterized by the occurrence of several fluorides: in addition to rosenbergite, elpasolite, and hydrokenoralstonite, fluorite, CaF2, and gearksutite, CaAlF4(OH)⋅ H2O, have been reported (Menchetti and Batoni, 2015). Along with these phases, recently the new mineral species dacostaite, ideally K(Mg2Al)[Mg(H2O)6](AsO4)2F6⋅ 2H2O, was described (Biagioni et al., 2024). Finally, EDS analyses performed on alunite revealed detectable F contents, with a simplified formula close to KAl3(SO4)2[(OH)5F]Σ6.00 (Cristian Biagioni, unpublished data), in accordance with Bayliss et al. (2010), who suggested the possibility of the occurrence of minor F in alunite supergroup minerals.
Even if the actual relations among these species have not been elucidated yet, they are probably the result of the late-stage circulation of (Al,F)-rich hydrothermal fluids within the fractures of silicified limestone hosting the Sb ore deposit. These fluids were probably also enriched in alkali and alkaline earth metals (Na, K, Mg, and Ca), favouring the crystallization of the fluoride minerals so far identified in the Stanza Santoni area.
Whereas the occurrence of fluorite is reported from several Sb ore deposits from Tuscany (e.g. Catabbio – Fornaseri, 1947; San Martino sul Fiora – Zucchetti, 1956; Pereta – Dessau and De Stefanis, 1969), the rare fluoride minerals reported from the Cetine di Cotorniano mine are a unicum among Tuscan Sb ore deposits. Only at the Pereta mine, Menchetti and Sabelli (1981) reported the occurrence of two other rare aluminium fluorides, minyulite KAl2(PO4)2F⋅ 4H2O, and fluellite, Al2(PO4)F2(OH)⋅ 7H2O, along with gearksutite, whereas Nannoni and Capperi (1998) hypothesized the occurrence of minyulite at Micciano. This latter finding is not supported by any analytical data and should be considered questionable.
Nannoniite is a new aluminium hydroxy fluoride showing structural relationships with gibbsite. Its study allowed the full characterization of the unnamed mineral UM1990-28-OHF:Al (Jambor et al., 1990).
Even if F is usually hosted in some rock-forming minerals like fluorite or apatite-supergroup compounds or in some hydroxy-silicates (e.g. Fuge, 2019), where F replaces (OH) due to their similar ionic size (Shannon, 1976), the identification of nannoniite suggests a possible role played by Al hydroxides in hosting this halogen. Finally, the solution of its crystal structure, made possible by the development of 3DED, highlights that the homovalent substitution (OH) F− has a deep impact on the arrangement of H atoms within the structure, whose expression is represented by the subtle differences in the X-ray powder diffraction patterns of nannoniite and gibbsite.
The Crystallographic Information File data of nannoniite is available in the Supplement.
The supplement related to this article is available online at: https://doi.org/10.5194/ejm-36-1011-2024-supplement.
CB, DM, and SM collected preliminary data. CB carried out single-crystal X-ray diffraction. EM and SL carried out electron diffraction studies. ND carried out the synchrotron experiment. DB and CB discussed the possible genesis of nannoniite. JS and ZD performed electron microprobe analysis. CB wrote the paper with input from the other authors.
At least one of the (co-)authors is a member of the editorial board of European Journal of Mineralogy. The peer-review process was guided by an independent editor, and the authors also have no other competing interests to declare.
Publisher’s note: Copernicus Publications remains neutral with regard to jurisdictional claims made in the text, published maps, institutional affiliations, or any other geographical representation in this paper. While Copernicus Publications makes every effort to include appropriate place names, the final responsibility lies with the authors.
This article is part of the special issue “New minerals: EJM support”. It is not associated with a conference.
Massimo Batoni is acknowledged for providing us with the specimens of nannoniite used for this mineralogical investigation.
This research has been supported by the Ministry of Culture of the Czech Republic (long-term project DKRVO 2024-2028/1.II.a; National Museum, 00023272) fo Jiří Sejkora and Zdeněk Dolníček.
This paper was edited by Sergey Krivovichev and Cristiano Ferraris and reviewed by Giovanni Ferraris and one anonymous referee.
Amici Mineralogisti Fiorentini: Ricordo di Valerio Santoni, Riv. Mineral. Ital., 16, 186, 2002.
Balan, E., Lazzeri, M., Morin, G., and Mauri, F.: First-principle study of the OH-stretching modes of gibbsite, Am. Mineral., 91, 115–119, 2006.
Balan, E., Blanchard, M., Hochepied, J.-F., and Lazzeri, M.: Surface modes in the infrared spectrum of hydroux minerals: the OH-stretching modes of bayerite, Phys. Chem. Miner., 35, 279–285, 2008.
Bayliss, P., Kolitsch, U., Nickel, E. H., and Pring, A.: Alunite supergroup: recommended nomenclature, Mineral. Mag., 74, 919–927, 2010.
Belluomini, G., Fornaseri, M., and Nicoletti, M.: Onoratoite, a new antimony oxychloride from Cetine di Cotorniano, Rosia (Siena, Italy), Mineral. Mag., 36, 1037–1044, 1968.
Biagioni, C., Mauro, D., Sejkora, J., Dolníček, Z., Dini, A., and Škoda, R.: Dacostaite, IMA 2024-015, in: CNMNC Newsletter 80, Eur. J. Mineral., 36, 599–604, 2024.
Bolokhontseva, S. V., Baturin, S. V., Ilmenev, E. S., Panova, M. A., and Purusova, S. P.: Zharchikhite AlF(OH)2, a new mineral, Z. Vses. Minerral. Obsh., 117, 79–83, 1988.
Bosmans, H. J.: Unit cell and crystal structure of nordstrandite, Al(OH)3, Acta Crystallogr., B26, 649–652, 1970.
Brese, N. E. and O'Keeffe, M.: Bond-valence parameters for solids, Acta Crystallogr., B47, 192–197, 1991.
Brizzi, G., Ciselli, I., and Santucci, A.: Ultime novità da le Cetine di Cotorniano (SI), Riv. Mineral. Ital., 9, 145–155, 1986.
Brown, I. D.: On the geometry of O–H O hydrogen bonds, Acta Crystallogr., A32, 24–31, 1976.
Brown, I. D. and Altermatt, D.: Bond-valence parameters obtained from a systematic analysis of the Inorganic Crystal Structure Database, Acta Crystallogr., B41, 244–247, 1985.
Burla, M. C., Caliandro, R., Carrozzini, B., Cascarano, G. L., Cuocci, C., Giacovazzo, C., Mallamo, M., Mazzone, A., and Polidori, G.: Crystal structure determination and refinement via SIR2014, J. Appl. Crystallogr., 48, 306–309, 2015.
Clark, G. R., Rodgers, K. A., and Henderson, G. S.: The crystal chemistry of doyleite, Al(OH)3, Z. Kristallogr., 213, 96–100, 1998.
Cliff, G. and Lorimer, W.: The quantitative analysis of thin specimens, J. Microscopy, 103, 203–207, 1975.
Dessau, G. and De Stefanis, A.: Studio geologico-minerario della zona mercurifera di Cerreto Piano (Scansano, Grosseto), Mem. Soc. Geol. It., 8, 289–324, 1969.
Dini, A.: Ore deposits, industrial minerals and geothermal resources, Per. Mineral., 72, 41–52, 2003.
Ferraris, G. and Franchini-Angela, M.: Survey of the geometry and environment of water molecules in crystalline hydrates studied by neutron diffraction, Acta Crystallogr., B28, 3572–3583, 1972.
Fornaseri, M.: Fluorite del Fosso “La Fuliggine” presso Catabbio (Grosseto), Per. Mineral., 16, 129–130, 1947.
Frost, R. L., Kloprogge, J. T., Russell, S. C., and Szetu, J. L.: Vibrational spectroscopy and dehydroxylation of aluminum (oxo)hydroxide: gibbsite, Appl. Spectrosc., 53, 423–434, 1999.
Fuge, R.: Fluorine in the environment, a review of its sources and geochemistry, Appl. Geochem., 100, 393–406, 2019.
Gatta, D. G., Nestola, F., Bromiley, G. D., and Loose, A.: New insights into crystal chemistry of topaz: A multi-methodological study, Am. Mineral., 91, 1839–1846, 2006.
Gemmi, M., Mugnaioli, E., Gorelik, T., Kolb, U., Palatinus, L., Boullav, P., Hovmöller, S., and Abrahams, P. A.: 3D Electron Diffraction: The nanocrystallography revolution, ACS Centr. Sci., 5, 1315–1329, 2019.
Huneke, J. T., Cramer, R. E., Alvarez, R., and El-Swaify, S. A.: The identification of gibbsite and bayerite by laser Raman spectroscopy, Soil Sci. Soc. Am. J., 44, 131–134, 1980.
Jambor, J. L., Sabina, A. P., Ramik, R. A., and Sturman, B. D.: A fluorine-bearing gibbsite-like mineral from the Francon quarry, Montreal, Quebec, Can. Mineral., 28, 147–153, 1990.
Kasatkin, A. V., Plášil, J., Chukanov, N. V., Škoda, R., Nestola, F., Agakhanov, A. A., and Belakovskiy, D. I.: Gurzhiite, Al(UO2)(SO4)2F⋅ 10H2O, a new uranyl sulfate mineral with a chain structure from the Bykogorskoe deposit, Northern Caucasus, Russia, Mineral. Mag., 86, 412–421, 2022.
Kolb, U., Gorelik, T., Kubel, C., Otten, M. T., and Hubert, C.: Towards automated diffraction tomography: Part I–Data acquisition, Ultramicroscopy, 107, 507–513, 2007.
Kraus, W. and Nolze, G.: Powder Cell – a program for the representation and manipulation of crystal structures and calculation of the resulting X-ray powder patterns, J. Appl. Crystallogr., 29, 301–303, 1996.
Lattanzi, P.: Epithermal precious metal deposits of Italy – an overview, Miner. Dep., 34, 630–638, 1999.
Laugier, J. and Bochu, B.: CELREF: Cell parameters refinement program from powder diffraction diagram, Laboratoire des Matériaux et du Génie Physique, Ecole Nationale Supérieure de Physique de Grenoble (INPG), Grenoble, France, 1999.
Mandarino, J. A.: The Gladstone-Dale relationship, Part III. Some general applications, Can. Mineral., 17, 71–76, 1979.
Mandarino, J. A.: The Gladstone-Dale relationship, Part IV. The compatibility concept and some application, Can. Mineral., 19, 441–450, 1981.
Mauro, D., Biagioni, C., Pasero, M., and Zaccarini, F.: Crystal-chemistry of sulfates from the Apuan Alps, Tuscany, Italy. VIII. New data on khademite, Al(SO4)F(H2O)5, Mineral. Mag., 84, 540–546, 2020.
Mauro, D., Biagioni, C., Sejkora, J., Dolníček, Z., and Škoda, R.: Batoniite, [Al8(OH)14(H2O)18](SO4)5⋅ 5H2O, a new mineral with the [Al8(OH)14(H2O)18]10+ polyoxocation from the Cetine di Cotorniano Mine, Tuscany, Italy, Eur. J. Mineral., 35, 703–714, https://doi.org/10.5194/ejm-35-703-2023, 2023.
Megaw, H. D.: The crystal structure of hydrargillite, Al(OH)3, Z. Kristallogr., 87, 185–204, 1934.
Menchetti, S. and Batoni, M.: Le Cetine di Cotorniano, Miniera & Minerali, Associazione Micro-mineralogica Italiana, Cremona, 353 pp., ISBN 978-88-905541-3-1, 2015.
Menchetti, S. and Sabelli, C.: Minyulite, associated with fluellite, from Pereta, Tuscany, Italy, N. Jahr. Mineral. Monat., 1981, 505–510, 1981.
Mugnaioli, E. and Gemmi, M.: Single-crystal analysis of nanodomains by electron diffraction tomography: Mineralogy at the order-disorder borderline, Z. Krist.-Cryst. Mater., 233, 163–178, 2018.
Mugnaioli, E., Gorelik, T., and Kolb, U.: “Ab initio” structure solution from electron diffraction data obtained by a combination of automated diffraction tomography and precession technique, Ultramicroscopy, 109, 758–765, 2009.
Nannoni, R. and Capperi, M.: I minerali della Sorgente Solfurea di Micciano, Riv. Mineral. It., 22, 61–65, 1998.
Olmi, F. and Sabelli, C.: Brizziite, NaSbO3, a new mineral from the Cetine mine (Tuscany, Italy): description and crystal structure, Eur. J. Mineral., 6, 667–672, 1994.
Olmi, F., Sabelli, C., and Trosti-Ferroni, R.: Rosenbergite, AlF[F0.5(H2O)0.5]4⋅ H2O, a new mineral from the Cetine mine (Tuscany, Italy): description and crystal structure, Eur. J. Mineral., 5, 1167–1174, 1993.
Palatinus, L., Brázda, P., Jelínek, M., Hrdá, J., Steciuk, G., and Klementová, M.: Specifics of the data processing of precession electron diffraction tomography data and their implementation in the program PETS2.0, Acta Crystallogr., B75, 512–522, 2019.
Pedersen, B.: The geometry of hydrogen bonds from donor water molecules, Acta Crystallogr., B30, 289–291, 1974.
Petříček, V., Dušek, M., and Palatinus, L.: Crystallographic computing system JANA2006: general features, Z. Kristallogr., 229, 345–352, 2014.
Pouchou, J. L. and Pichoir, F.: “PAP” (φρZ) procedure for improved quantitative microanalysis, in: Microbeam Analysis, edited by: Armstrong, J. T., San Francisco Press, San Francisco, 104–106, 1985.
Robbins, M.: Fluorescence: Gems and minerals under ultraviolet light, Geoscience Press, Phoenix, 384 pp., ISBN-10 094500513X, ISBN-13 978-0945005131, 1994.
Rodgers, K. A.: Routine identification of aluminium hydroxide polymorphs with the laser Raman microprobe, Clay Miner., 28, 85–99, 1993.
Ruan, H. D., Frost, R. L., and Kloprogge, J. T.: Comparison of Raman spectra in characterizing gibbsite, bayerite, diaspore and boehmite, J. Raman Spectrosc., 32, 745–750, 2001.
Saalfeld, H. and Wedde, M.: Refinement of the crystal structure of gibbsite, Al(OH)3, Z. Kristallogr., 139, 129–135, 1974.
Sabelli, C. and Vezzalini, G.: Cetineite, a new antimony oxide-sulfide mineral from Cetine mine, Tuscany, Italy, Neues Jb. Miner. Monat., 1987, 419–425, 1987.
Shannon, R. D.: Revised effective ionic radii and systematic studies of interatomic distances in halides and chalcogenides, Acta Crystallogr., A32, 751–767, 1976.
Sillitoe, R. H. and Brogi, A.: Geothermal systems in Northern Apennines, Italy: modern analogues of Carlin-style gold deposits, Econ. Geol., 116, 1491–1501, 2021.
Smith, D. G. W. and Nickel, E. H.: A system for codification for unnamed minerals: report of the Subcommittee for Unnamed Minerals of the IMA Commission on New Minerals, Nomenclature and Classification, Can. Mineral., 45, 983–1055, 2007.
Zigan, F., Joswig, W., and Burger, N.: Die Wasserstoffpositionen im Bayerite Al(OH)3, Z. Kristallogr., 148, 255–273, 1978.
Zubkova, N. V., Pekov, I. V., Ksenofontov, D. A., and Pushcharovsky, D. Y.: Zharchikhite, AlF(OH)2: a novel structure type related to α-PbO2, Acta Crystallogr., B80, 38–41, 2024.
Zucchetti, S.: Il giacimento antimonifero di San Martino sul Fiora (Grosseto), L'Ind. Miner., 7, 539–544, 1956.